1. Introduction
The phylum Apicomplexan is made of a sizeable set of eukaryotes that cause various diseases in animals and humans. This phylum is composed of obligate eukaryotic cells that are part of the Chromalveolata. They have apical complexes at their end, additionally, some of them also retain a non-photosynthetic plastid, which is referred to as the apicoplast. Various branches of the Apicomplexa reside in distinct ecological niches with differing metabolic abilities. Most of the time, Apicomplexa are motile, and the smooth gliding of the Apicomplexa is carried out through a mechanism that uses small static myosin motors and adhesions (1). The smooth gliding capacity enables the parasite to navigate across substrates, migrate, invade tissues, then egress from one infected cell to another susceptible cell (2). The apical complex of Apicomplexa are structures and secretory organelles which consists of various components that are required for the invasion of host cells during the various stages of the life cycle of the organism (3). The life cycle of Apicomplexa is typically complex. There are four transformations that occur throughout the life cycle, including the sporozoite, the zygote, the merozoite, and the gametic stages. The typical lifecycle of Apicomplexa involves multiple sexual and asexual reproduction events involving multiple hosts (4). In the meiotic division, the sporozoite forms into infective haploid sporozoites. They are known to target specific cells within the body using a variety of molecular armaments. These include the binding of antigens to host cells and the surface adhesion of surface proteins. The sporozoites change into merozoites when they enter the host cells. After several generations of asexual division, the sporozoites emerge to produce more merozoites. The development of a diploid zygote then leads to fertilization and the establishment of infective diploid sporozoites (5).
Apicomplexans are known to be auxotrophic for various types of lipids. Thus, they forage for lipids such as cholesterol and sphingolipids from their host utilizing their unique lipid-transporter systems. The Apicomplexans have lipids that differ from the lipids of their hosts (6). For instance, they possess lipid biosynthetic pathways, such as the prokaryotic methylerythritol phosphate pathway that is in control of making isoprenoid precursors, which are uniquely Apicomplexans (7). In order to store fats, such as cholesteryl acetate, the Apicomplexans can use their unique enzymes. Although this uniqueness confers competitive advantage for the parasite, such parasite-specific pathways can also serve as crucial goals for the advancement and production of selective drugs. Blocking cholesterol esterification for instance is known to kill T. gondii. Fosmidomycin, is one such drug used against malaria (8). Therefore, these pathways are useful in the development of new drug targets. Based on recent genome data and biochemical studies, this review paper will analyze the diversity of metabolic flow of calcium ions, distribution of Apicomplexans in particular Plasmodium and Cryptosporidium. Plasmodium and Cryptosporidium have strong ability to occupy diverse niches and their metabolic pathways are expected to be different according to their survival mechanism in the niche they occupy. This review also discusses the various unique Apicomplexans pathways in selected Apicomplexans that have public health importance and highlights their potential as therapeutic targets.
1.1. General Characterization of Plasmodium spp
Plasmodium, the malaria parasite, is one of many Apicomplexans that are blood-borne parasites of terrestrial vertebrate and transmitted by biting insects. In humans, malaria is transmitted by the anopheles’ mosquito. Five distinct species of Plasmodium infect humans and produce somewhat different disease scenarios. Around 300 to 500 million incidents of malaria are recorded annually in the tropics, and around 1.1 to 2.7 million deaths are reported each year (9). The hematological alterations that can be caused by the infection vary depending on the degree of immunity, the host genetic elements, and the parasites' strains. Majority of the mortality cases are due to Plasmodium falciparum infection (10). Like for the most part of Apicomplexan parasites, the life succession of Plasmodium is sexual, involving three distinct events of reproduction, each potentially involving productions of many progenies through binary fission (11). During this event the parasite will produce merozoites and gamogony, which will differentiate to male gametes. At the same they produce sporogony, which is meiotic and produce sporozoites (4). The stage transmitted by the mosquitoes is the sporozoites. Sporozoites enters the blood stream and invade liver cells. In the liver they undergo merogony again. When the erythrocytes burst, the emerging merozoites are free to invade other cells and this cycle then repeats itself. When an infected human is bitten by another mosquito, gametocytes are engulfed into the gut of the insect, and develop into female gametes, or undergo gamogony and produce male gametes (5). Gametes forming a zygotic cell are the only diploid stage. Diploid cells invade intestinal cells of the mosquito and undergo sporogony to produce haploid sporozoites, which enter the salivary glands, and awaits delivery into another person for the infection to continue (12–14).
2. General Characterization of Cryptosporidium spp
Cryptosporidium parasite causes a diarrheal illness known as cryptosporidiosis. Most people in developing countries, especially immunodeficient individuals and children, the prevalence of cryptosporidiosis is significantly higher. Despite the immense burden of this disease, no effective treatment or prevention is available (15). This parasite has been known to affect various animals and humans (16). Unlike Plasmodium, which can be transmitted through a mosquito, Cryptosporidium does not rely on a vector for its transmission. Instead, it can achieve its full life cycle in a single host (17). This allows it to infect other people and thus can easily be transmitted to new hosts. Several Cryptosporia infect mammals. C. parvum, C. canis, C. felis, C. meleagridis, and C. muris causes diseases in humans. Typically, Cryptosporidium infection does not last long, however can become serious and deadly in infants and persons with weakened immunity. In an infected individual, the parasite persists in the colon for approximately 5 or more weeks (18). This parasite can be spread by oocysts that are produced when a person ingests the infested substance. They then develop an infection in the small intestine. The entire genetic constitution of the C. parvum has been sequenced as early as 2004, was discovered to be different from other eukaryotes, they found that its mitochondria do not have DNA. The genome information of this organism is available through the database, CryptoDB.org (19–21).
3. Calcium Signaling
Calcium-mediated signaling is used by Apicomplexans parasites to control a range of vital events, such as the secretion of proteins and the movement of cells, and this signaling process is carried out through a variety of specialized systems. These systems are noted to differ from their hosts (22). A comparative analysis of the genome of three of the most important parasites of Apicomplexans (Toxoplasma gondii, Plasmodium spp. and Cryptosporidium spp.) revealed that they can adapt to the effects of calcium on their cellular responses. For instance, the Apicomplexans can produce a variety of P-type Ca2+ ATPases involved in the ER-type reuptake process (23–25). Besides these, the parasites also have genes that are linked to the Golgi PMR-like calcium channels and the Ca2+/H+ exchanger. In contrast, the plasma membrane-type Ca2+ ATPases are only found in the T. gondii (26). The presence of calcium in Apicomplexans is known to control various processes, such as protein secretion and energy production, motility, differentiation etc. (26). Tricarboxylic acid is a vital component of the energy production process in prokaryotic and eukaryotic organisms. However, different studies have revealed that the enzymes that are used in this process are different in each species. Studies have shown that the occurrence of calcium in the Apicomplexans performs a significant role in this process (27).
3.1. The Calcium ATPase Pump
The SERCA pump is a vital component of the regulation and transport of calcium in the cellular environment. It allows the transportation of nutrients from the cytosol to the reticulum. In vertebrates, the three SERCA genes are shown to have different spliced forms. Apicomplexans have a single Ca
2+ ATP enzyme, which is required for the production of calcium. The pumps of SERCA are responsible for filling the endoplasmic reticulum with calcium which is the sources of the readily available and mobilizable source of calcium signaling (28). The genome of the Apicomplexans group has revealed new information about the mechanisms by which calcium production is regulated in living organisms. Although mitochondria are important in the development of organisms,
Plasmodium does not have mitochondrial pyruvate dehydrogenase and H+ translocating NADH dehydrogenase (Complex I, NDHI) (29). In addition,
Plasmodium does not have a significant amount of mtDNA. This makes it vulnerable to oxidative phosphorylation during its life cycle. Alternatively, other
Cryptosporidium species, e.g.,
Cryptosporidium parvum and the
Cryptosporidium hominis, lack certain enzyme components, such as pyruvate dehydrogenase. In order to maintain its function, they can be substituted for by other enzymes, such as malate-quinone oxidoreductase (MQO) (as shown in
Figure 1), alternative oxidase (AOX), and pyruvate-NADP
+ oxidoreductase (PNO) (29).
Calcium is an essential additional courier in animal cells (21,30). Compared to the extracellular environment, the level of calcium in the cell is kept at a thousand-fold lower in the cytosol. This is due to the rapid release and influx of this substance, which is linked to various functional reactions. Calcium flow into cells via a range of pathways, including the plasma membrane and the secretory pathway (20,31). The endoplasmic reticulum is a major reservoir of calcium. The SERCA pump is Ca2+ ATPase and is located in the sarcoplasmic reticulum. In animal cells, this enzyme is activated by the IP3 channels (19). The ryanodine receptor is a component of the second structure that permits rapid release of calcium from the endoplasmic reticulum. It is also involved in the release of calcium from the sarcoplasmic reticulum (32). Specialized receptors for IP3 (IP3R) and ryanodine (RyR)-mediated calcium receptors which mediate calcium release have evolved during differentiation of vertebrates (33). It has been shown that plants and protozoa are also responding to the agonists of these intracellular calcium channels. Studies on animals have shown that calcium release channels, which are involved in these responses are not conserved among animal species.
Calcium regulation in the protozoan parasite is controlled by a number of organelles such as the ER, mitochondria, and acidocalcisomes (34). Acidocalcisomes stores calcium in large amounts. They are acidic stores which are known to be conserved in several living things. Acidocalcisomes are crucial in the metabolism of polyphosphate (35). Calcium controls differentiation (20), motility (36), secretion (31,37), and many other crucial phenomena in the Apicomplexan parasites. In the tricarboxylic acid (TCA) cycle of these eukaryotic parasites, calcium is a major player, serving as a crucial regulator of the cycle.
The TCA cycle utilizes several enzyme-catalyzed reactions. All organisms that utilizes oxygen for respiration run their cellular respiration via the TCA cycle. In the mitochondria of eukaryotes, the citric cycle operates in the matrix. Anaerobic organisms utilize the citric acid cycle to convert various nutrients into usable energy. This process involves the conversion of fats, proteins, and carbohydrates into water and carbon dioxide. Among the reactions that are relevant to this process are pyruvate oxidation and glycolysis. These occur before and after the citric acid cycle. The TCA cycle is important for the synthesis of amino acids. It provides various amino acid precursors (30,38,39).
The product inhibition and substrate availability of the TCA cycle are the most important factors that affect its regulation. Pyruvate can be used to produce acetyl-CoA by glycolysis, amino acid metabolism, and fatty acid oxidation. Different fates for this chemical exist. It can also be used to increase the reaction rate of various steps in the cycle. This helps in maintaining a steady flux throughout the process. Feedback inhibition is also performed by citrate when it affects the activity of the phosphofructokinase, which is an enzyme involved in the process of glycolysis. This prevents a steady increase in the flux due to the accumulation of citrate and the decreased substrate availability. Studies on hypoxia-inducible factors (HIF) have thrown more light into the mode of operation of the citric cycle. Recent studies have shown that the interaction between the citrate intermediates and HIF is related to the regulation of various processes, such as ion transport and oxygen homeostasis. The catalytic activity of the ubiquitin ligase complex is also regulated by the interaction between the proline residues and the E3 complex. The interaction between the E3 complex and the proline residues of HIF is also regulated by the cyclic hydroxylation which effects their ubiquitination and subsequent degradation. The catalytic action of this reaction is carried out by the prolyl-4-hydroxylases. The use of succinate and fumarrate has been identified as effective inhibitors of these prolyl hydroxylases (33,40–45).
The process of fatty acid biosynthesis involves the conversion of Acetyl-CoA precursors to long acyl chains. These chains usually contain 8 or more carbon atoms. All organisms have the same reaction mechanisms when it comes to fatty acid synthesis. They can be split into an elongate cycle and an inhibition phase. The initial step in the process of fatty acid synthesis involves the transfer of malonyl-CoA to the acyl carrier protein. This protein then anchors the growing acyl chain. The transfer of another Acyl-COA moiety to the malonyl acid-ACP completes the initiation step. This process involves performing four steps. The product of this step, which is -Ketobutyl-ACP, is then reduced to β-Hydroxyl-ACP, enoyl-ACP, and finally to acyl-ACP. The addition of malonyl to the acyl chain during a condensation reaction activates the acyl-ACP cycle. The continuous cycle of this process involves adding two carbons to the acyl chain until it reaches its full length. The resulting reaction can be terminated by either the transesterification or hydrolysis of the fatty acyl chain (46–51).
Most eukaryotes have membrane-bound organelles known as mitochondria, which are responsible for producing ATP in aerobic species. They have an outer membrane and an inner membrane that are called cristae. The cristae is an important site in the TCA cycle, and the mitochondrion uses a combination of acytyl-coA as substrate. The mitochondrial membranes are energy-transmitting membranes. They hold the enzymes for the electron transport chain that leads to ATP synthesis, using oxygen as the last electron acceptor. Although mitochondrion is an essential organelle for energy production however, many intestinal parasites and symbionts no longer have mitochondria because the anaerobic habitat does not provide sufficient oxygen for the electron transport chain reactions. The three main types are tubular, flattened, and discoid. It is one of the morphological features that has been useful to understand the relationships among protists (37,52–54).
The mitochondrion is an unusual organelle of eukaryotes, and its acquisition was explained by endosymbiosis (19,20). New discoveries in the area of phylogenetic analysis, including the identification of mitochondria in several species, such as Entamoeba, Giadia, and Parabasalia (38,45,55,56). It has been suggested that there is no ancestrally branching lineage of eukaryotes. The importance of the mitochondria is also apparent in other species. Most matrix proteins that are derived from the mitochondria have an NH2-terminal presequence, which is removed by a matrix protease after it has been transported across the membrane. Although the data from this sequence did not contain a common amino acid structure, it can still form an amphiphile-helix (50). Although it is believed that the import protein from the mitochondria is conserved in plants and animals, there are differences. For instance, the plant mitochondria target presequences with a higher serine constant (57). It has been suggested that the plant's organelle targets different presequences due to the presence of nuclease-encoded genes for the chloroplast proteins. This suggests that the accuracy of the prediction of presequences from the genome of plants is less (58).
3.2. Diversity of Calcium Channels among Cryptosporidium and Plasmodium spp
The calcium channel is a vital component of the Apicomplexan parasite's development and control. It plays a role in several important events, such as differentiation and secretion. The genome of the Apicomplexan parasite does not contain a family of calcium release channels that are found in metazoan animals (e.g., the IP3R channels) (59). Drosophilia and C. elegans possess only one IP3R calcium channel, while numerous replicas are existing in vertebrate. IP3R contains many domains including SPYR domain, which mediate protein-protein interactions. Even though two of the protein's SPYR domains are in the T. gondii, they do not contain motifs that are related to the calcium channel (30). Homologues for IP3 have not found in fungi, protozoa (39,47). The presence of a more competitive calcium channel in plants and protists suggests that the domains that are responsible for the IP3 channels also evolved in the evolution of metazoan species.
The members of the P-type ATPases are a group of ion pump-related proteins that are commonly found in eukaryotes, bacteria, and archaea. Most of them are focused on pumping a wide variety of cations. One of the subcomplexes of the group is involved in maintaining the biomembrane’s asymmetric nature by flipping phospholipids. Examples of the P-type cation-transporting ATPases are the potassium-potassium pump, the sodium pump, the proton pump, and the calcium pump. They use the energy from the hydrolysis of ATP to move cations across the membrane. The members of the SERCA pump family are responsible for replenishing the ER store. This is the source of calcium that is needed for signaling (48). Three SERCA genes are present in vertebrates. Each of these shows an alternative spliced form (51,57). Research demonstrates that the Apicomplexan parasite contains a one SERCA-type Ca2+ ATPase. This protein has been well-defined in P. falciparum (59). The discovery of the SERCA-type ATPase in the malaria parasite has led to the identification of a potential target for artemisinin (60), drugs that possess the most rapid action against P. falciparum malaria. Artemisinin involves in disruption of calcium homeostasis (13).
The domains of calcium influx channels are similar to those of the super family of ion channel structures. They include voltage-gated, cyclic nucleotide-gate, transient receptor potential, and other similar channels (14). Both voltage-gated (VG) and ligand-gated calcium channels are present in plants and animals, which are most commonly localized in the plasma membrane. In plants and animals, the voltage-gated and ligand-gated calcium channels are known to respond to membrane polarization changes to allow the selective influx of cations (41). Studies have shown that the VGCC can be found in ciliates (61). Several genes encoding the VGCC were identified in T. gondii. But the results of the genome-wide analysis revealed that the VGCC is not found in C. parvum or P. falciparum. The findings of the genome-wide analysis suggest that the VGCC is distributed randomly across the parasites. The analysis revealed that the Apicomplexans lack both glutamate- and cyclic nucleotide-gated calcium channels (36). The cyclic nucleotide-gated cation channel is similar to the voltage-gated family of channels in structure. It also opens in the presence of certain cyclic nucleotides. The negative and positive charged regions of the cyclic nucleotide-gate channels are often involved in membrane potential changes. The glutamate-gated channels, on the other hand, are closed or opened when a chemical messenger binds to them.
4. Carbohydrate and Energy Production among Plasmodium and Cryptosporidium spp
One of the ways to obtain energy is through respiration. This process involves breaking down the six-carbon glucose into smaller molecules. Both animals and plants have respiratory reactions that are similar to those of glycolysis. The Krebs cycle is for aerobic organisms, while the glycolysis cycle is for all living creatures. The rate at which carbon dioxide and oxygen consumption are produced or consumed can be measured during respiration. Both require the help of various enzymes to catalyze their reactions. The electron transport chain is one of the most important features of respiration. The Krebs cycle involves the accumulation of NADH in the mitochondrion, and in the presence of oxygen, the transport chain activity is carried out in the inner membrane of the mitochondrion (39,62).
There are two pathways that allow living organisms to breathe: the common pathway, which is known as cytochrome respiration, and the alternative pathway, which is commonly utilized by plants, fungi, and some other organisms. The alternative pathway is different from the cytochrome respiration route in that it goes through a different branch point. The latter is typical for living organisms. As complex III pulls another proton from the matrix, cytochrome C grabs electrons and moves toward the inner membrane's outer side. As complex IV pulls another proton from the matrix, it transfers the electrons to the mitochondrion's inner domains. At this stage, the two electrons yield water, and the proton is used to consume oxygen. Although it oxidizes NADH to its negative state, it does not contribute positively to the proton gradient.
The blood-stage parasite Plasmodium ferments glucose as its primary source of energy. The process of glycolysis, which involves converting glucose to lactic acid, is similar to that of other organisms. The activity of the various enzyme genes in Plasmodium has also been identified. The Plasmodium parasite has a high level of glycolysis. It can utilize up to 75 times the glucose of uninfected cells. The high level of LDH activity is believed to be responsible for the regeneration of the NAD+ molecule. The energy currency of a cell is derived from glycolysis, which results in the production of ATP. ATP is required for homeostatic and anabolic processes.
Most of the glucose that the parasites use is converted to lactic acid. However, some of these intermediates can be diverted to other uses. For instance, the pentose phosphate pathway can provide ribose sugars that are required for nucleotide metabolism. This pathway could also help in the regeneration of NADPH. Further pyruvate metabolism can provide intermediates in various biological pathways (38,46,63).
The process of aerobic metabolism involves catabolizing pyruvate and carbon dioxide to form hydrogen and then reducing the NAD+ to the NADH level. The electrons then get transferred to molecular oxygen and are then produced water. The resulting ATP is produced through the process of oxidative phosphorylation. The combined effects of the TCA cycle and the oxidation process can yield up to 38 ATP molecules for each glucose molecule. On the contrary, glycolysis only produces around two ATP molecules for every glucose molecule. The metabolic efficiency of malaria parasites can be attributed to huge presence of glucose in the blood of it’s host (33,40–44).
Oxidation phosphorylation and the TCA cycle are typically carried out in the eukaryotes' mitochondria. These processes are believed to be nonfunctional in the parasites' blood-stage (64). Research data now shows that the parasites’ blood-stage metabolism can also benefit from the development of an electron transport chain (65–67). Inhibition of the transport of electrons by atovaquone can also cause the membrane potential of the parasites to collapse (21,68).
Apicomplexans are known to occupy diverse niches within the cell. They have remolded their mitochondria through the evolution of a reductive metabolic process. Plasmodium, on the other hand, lacks a pair of catalytic subunits (i.e., the mitochondrial pyruvate dehydrogenase and H+ translocating NADH dehydrogenase). The mitochondrion has minimal mitochondrial DNA, and the mitochondria is put to use in oxidation at the insect stage (NDH2). Parasites rely on glycolysis to generate energy in the blood of mammals, however at this stage the mitochondria are downregulated. In the metabolisms of human intestine parasites, the cryptosporidium parvum and cryptosporidium hominis lack a variety of catalytic subunits. Mitosomes of the rodent gastric parasite Cryptosporidium muris retain all of the cycle’s enzymes oxidoreductase and the unique respiratory chain. Cryptosporidium mitosome use AOX, MQO, and PNO.
The MQO of all the Apicomplexan parasites and the dinoflagellates is acquired from epsilon proteobacteria through a lateral gene transfer. The absence of mitochondria in C. parvum has been a controversial issue. Early studies suggested that this organelle could not be found in the sporozoites, and an analysis of the structures of the sporozoite samples did not reveal any similar structures to those found in other Apicomplexans. Most biochemical studies indicate that C. parvum has an anaerobic metabolism. However, it is possible that the morphological changes that occur during differentiation can make it difficult to visualize the changes in the metabolic function and form of the mitochondrion.
In contrast, the structures of the mitochondria in P. falciparum, which is a member of the Apicomplexan parasite, differ significantly. During the respiration of the mitochondria, the electrons are transferred to the ubiquinone, the cytochrome oxidase, and the complex IV. The protons are then transferred to the inner membrane, where they form a transmembrane potential. The cytochrome oxidase and the alternative pathway diverge from one another following the ubiquinone complex. The electrons then flow through the AOX, which is responsible for supplying water to the oxygen-producing mitochondria. Some plants also have additional AOX-like compounds known as immutans. The role of this enzyme in C. parvum's metabolic activity is still unclear. The AOX pathway cannot contribute to the transmembrane potential or the potential coupling sites of proton transport, which results in the loss of ATP production. It can function as an energy overflow device. It can also maintain a partial electron chain to allow the TCA cycle to continue. The AOX is produced by the alternative pathway, which diverges from the ubiquinone pool’s cytochrome-associated electron transport chain. Proton translocation is only performed at complex I, resulting in a lower ATP yield. The AOX is affected by various factors, such as cold and reactive oxygen species, as well as infection. The AOX can enhance the parasite’s ability to overcome oxygen species and diminish its level of oxidative stress. The pathway that allows T. brucei cellular respiration relies on the alternative oxidase. The metabolic differences between the human host and the parasite (T. Brucei) have made the alternative pathway a target for ascofuranone. The alternative oxidase' ability to bypass the electron transport chain's inhibition can also contribute to the development of resistance to fungicides by fungi. Some strobilurin fungicides, such as fluoxastrobin and azoxystrobin, can also prevent spore germination by reducing the alternative pathway's production of ATP (13,21,69,70).
5. Acidocalcisomes
The acidocalcisomes found in human cells and bacteria are made up of compounds that contain phosphorus, such as polyP, pyrophosphate, and orthophosphate. These acids are maintained by a combination of proton pumps, including the VP1 or V-H+ ATPase. Studies on trypanosomes and other species revealed that these structures play a role in water homeostasis, cation and phosphate metabolism, and autophagy. Their interactions some several organelles revealed some significant roles in osmoregulation and Ca2+ transports. Despite the presence of acidocalcisomes in organisms, they have been shown to interact with other organelles and have direct relevance to parasites. When first discovered in trypanosomes, acidocalcisomes, it was later discovered that these structures were similar to those found in bacteria, and they were referred to as volutin or metachromatic granules. The original inconsistency regarding the existence or nonexistence of membranes in these structures was not clear. During the twentieth century, some unicellular protists were shown to possess acidocalcisomes e.g., in trypanosomes, sarcosporidia, and coccidians. Although they were initially regarded as granules, they were eventually published as polyP bodies afterward research showed that these compounds were profuse in yeast's acalcisome-like gastrointestinal tract. Recently, it was discovered that polyP, a polymer that is a combination of orthophosphate and phosphohydride bonds, can be found in membrane-bounded calcium stores in human cells. These compounds were initially regarded as a type of acalcisome-like organelle. Studies show that acidocalcisomes and lipid droplets are membrane-bound organelles that are the only conserved organelles in human cells and bacteria.
Trypanosomes are known to contain acidocalcisomes that are frequently near other organelles (e.g., subpellicular membrane and mitochondria) (71). These close interactions could be relevant to the regulation of the cell's bioenergy levels. In studies conducted by Rizzuto et al, they noted that the presence of the IP
3R could trigger the release of Ca
2+ to the mitochondria (72). Acidocalcisomes are also close to the organelles that are situated near the mitochondria, which creates a high concentration of Ca
2+ in the region. The findings support the idea that the cell's acidocalcisomes and mitochondria have a close proximity (73) e.g., see
Figure 2. It has been suggested that a quasi-synaptic transmission of Ca
2+ between the acalcisomes and the mitochondria could occur. In addition, the effects of Ca
2+ on the carriers and the various cellular functions are proposed in trypanosomes. One of the targets that could be considered is the proline dehydrocarbamylase, which has an EF-hand domain. Another target is the pyruvate dehydrocarbamylase, which can be activated by a Ca
2+ dependent phosphatase (74).
Acidocalcisomes, are rich in phosphorus and calcium. Mammals seems not to have acidocalcisomes, moreover, acidocalcisomes serve as a large reservoir of Ca2+ than most intracellular pools (78,79). Fluorescent probes such as acridine orange can be used to identify acidocalcisomes because they produce a shift in fluorescence and absorption spectra when they accumulate in acidic compartments such as acidocalcisomes (80–82). T. cruzi, T. brucei, L. donavani, P knowlesi and T. gondii are all known to contain acidocalcisomes, and these specifically are also known to accumulate pyrophosphate that serves as an energy source for acidification of the compartments. Acidocalcisomes contains high amount of H+, the accumulation of this ion is via the vacuolar type H+-ATPase (83–85). The H+ pyrophosphatase in T. cruzi has been demonstrated in in vitro assays that they are sensitive to imidodiphosphate or aminomethylenediphosphate (AMDP) and bafilomycin A1 (86,87). It has been shown also that T. cruzi, T. gondii, P. falciparum, T. brucei, and L. amazonensis contains bafilomuycin-sensitive acidocalcisomes (88–90). Another pump of interest is the Na+/H+ exchanger which has also been demonstrated to be sensitive to 3,5-dibutyl-4-hydroxytoluene, however this pump is insensitive to ameloride (91–93).
The AMDP and bafilomycin A1 mode of action is the disruption of acidification of acidocalcisomes, they cause the neutralization of the acidic compartment and the release of calcium ions, since principally, the H+ gradient is a prerequisite condition for Ca2+ accumulation in these compartments (94–96). The amount of Ca2+ -ATPase differs in course of the parasite’s life cycle, for instance, an increased amount of acidocalcisomes in T. cruzi has been demonstrated in the amastigote form with a corresponding increased amount of calcium ions (97,98). Via 31P-NMR, it has been shown that P. falciparum, L. major, T. gondii, T. brucei, and T. cruzi contains long chains of phosphates bound to oxygen in acidocalcisome compartments (87,99,100). Interestingly, it has been demonstrated that the stage transition from trypomastigote to amastigote has been shown to possess significantly high long-chain polyphosphates in T. cruzi (101,102). This might serve as a plausible pathway to inhibit parasites with acidocalcisomes, for instance, researchers have been successful in using pyrophosphate analogs to limit the growth of T. cruzi, T. gondii, P. falciparum and Cryptosporidium parvum (103–106). Thus, the ubiquitous presence of acidocalcisomes in Apicomplexans is an indicator that they can serve as drug targets against these parasites.
Additionally, in Apicomplexan’s have enzymes that are different from those found in humans. These might be useful targets for chemotherapy. For instance, the pyrophosphatase that transport proton, and the soluble inorganic pyrophosphatase, can be blocked by pyrophosphate analogs (bisphosphonates). Renown Professors Docampo and Moreno pointed out that several drugs, such as diamidines and bisphosphonates, can accumulate in the acidocalcisomes. These drugs can either increase or decrease the number of these organelles. The mode of activity of these drugs is analogous to the prenyl diphosphate synthesis enzyme (107).
6. Diversity of Fatty Acid Synthesis among Apicomplexan spp
Although the fatty acid synthesis reactions of eukaryotes and prokaryotes share a common mechanism, their structures are different. In eukaryotes, for instance, malonyl-COA is synthesized by a combination of ACCase and a small carrier protein. The subsequent synthesis of fatty acid chains is carried out by a group of discrete enzymes known as Type II FAS. (108). ACCase is a multi-domain protein, while malonyl-CoA can be linked to the antacid component of a massive, multi-functional enzyme known as Type I FAS that performs the steps of the pathway (56). Plant species are hybrid when it comes to the synthesis of fatty acid. The plastid contains a type II FAS pathway, which is triggered by an endosymbiotic event. This pathway is the only one that leads to the de novo generation of fatty acids in plants (56). Like other eukaryotes, plants also have multi-domain ACCases in the cytoplasm. These allow for the production of malonyl-CoA and other metabolic pathways (35,109). Most plants also have multi-subunit ACCases in the plastid. These are similar to prokaryotic structures (109). The exemption being grasses belonging to the family poaceae, which have an ACCase similar to that of a eukaryotic cell (35,56). This unusual combination of enzymes is also present in the Apicomplexa.
Parasites that are known to be capable of exploiting two sources of fatty acids, namely, long chain fatty acids and triglycerides, are known to be the P. falciparum and T. gondii (35,109,110). The parasites are known to modify the fatty acids found in their host tissues (110), the inhibitors of these fatty acids converting enzymes have been demonstrated to possess anti-parasitic properties (12). The mechanisms by which the parasites modify the fatty acids and efforts to develop effective drugs against this process has been well documented (109). It has been previously reported that Plasmodium falciparum and T. gondii can also modify the fatty acids in their host cells. But, according to the genome data of the parasites, they only produce type II compounds (56). This finding validated the notion that the parasites can add radio-labeled acetate to their fatty acids (13).
It has been demonstrated that the apicoplast fatty acid pathway is a target of various Type II FAS inhibitors (109). The complete genome of P. falciparum has allowed the identification of all the required proteins for type II FAS in the organism's apicoplast. The sequence of the fatty acid synthesis pathway in the phylum has also become more complex, and the interactions between host and parasites are becoming more apparent. It is believed that some Apicomplexans have both type I and II FAS. Some families have only one pathway that is involved in the synthesis of fatty acid while the process has been discarded thoroughly by others (35,108). In Toxoplasma and Plasmodium, type II, apicoplast-localized synthesis pathway is used whereas in Cryptosporidium parvum, only type I FAS handles long chain fatty acids (35). In addition, Cryptosporidium parvum also has a type I FAS that is capable of producing polyketides. These are not found in other Apicomplexans (109).
The fatty acyl chain must be attached to the prosthetic 4'-phosphopantetherinyl moiety in order for the synthesis of fatty acid to proceed. On the other hand, newly synthesized domains or enzymes that lack this moiety are apoproteins that do not have the phosphopantetheinyl moiety. Transfer of the phosphopantetheinyl residue from the CoA to the serine residue in the AACP is performed by the PPTase. This process is specific to type II FAS in certain bacteria (35). ome of the surfactin production enzyme subclasses are specific to type I. Examples of these include the human PPTase and the yeast Lys5 (56,108,109). In addition to being a critical component precursors production for prokaryotic organisms, fatty acid synthesis is also crucial in the development of metabolic and intermediate processes. This process involves the conversion of Acetyl-CoAs into long acyl chains. Different species have their own reactions that involve these enzymes.
Mechanisms of Apicomplexans uptake of fatty acids
Literature suggest that scavenging of fatty acids in host cells by Apicomplexans have been examined (111). It has been demonstrated that the incorporation of fatty acids (radiolabeled amino acids) by parasites into their bodies happens during the incubation stage (112–114). In the case of
T. gondii, this process occurs in free tachyzoite parasites. They then transform fatty acids from the host into triacylglycerides. These parasites then accumulate these triglycerides in their bodies. The DGAT enzyme is utilized to convert fatty acids into triacylglycerides (115,116). Different species of Apicomplexans live in host cells that have different metabolic potentials and architectures. It is possible that the various cellular mechanisms that facilitate the uptake of fatty acids exist. The various Acyl-CoA binding proteins and Acyl-CoA synthases that are encoded by the Apicomplexa are involved in the transport and salvage of fatty acids especially in
P. falciparum (117), C. parvum produces a single acyl-coA binding protein. This component includes an ankyrin and a binding domain. It also favorably associates palmitoyl-coA, the precursor of PKS and FASI (118). This protein, together with
C. parvum's oxysterol binding component, has been shown to be a component of the lipid salvage process in the host cell (110,119). In Toxoplasma, it has been observed that the PV employs a close relationship with the host cell's mitochondria and endoplasmic reticulum (120), this findings relates to this protein in the liver stage of
Plasmodium (121). The importance of this close interaction has been shown to be in the uptake of lipid products by bacterial pathogens (122). Another study has shown that the secretory pathway of the endosome/lysosome is linked to the host cell's lipid salvage process. This finding was based on the studies that revealed that intracellular parasites can acquire sterols from their medium (123,124). A study conducted by Coppens et al. revealed that the microtubular projections of the host cell are transported to the PV's space. These structures are then trapped in the cavity of the vacuolar membrane, where GRA7 is involved (125), see
Figure 3.
The study also noted that the PV's lysosomes could serve as a conduit for other lipid-related activities which contradicts the conventional opinion that PVs are dysfunctional and should be secluded from the endosomes and the secretory pathways. Studies on the uptake of fatty acids by Plasmodium are needed to develop a more accurate model. The most recent study focused on the expression profiling of Plasmodium's genes during the life cycle. The work revealed that many of these genes are upregulated in the infective sporozoite (126–128). Studies targeting these genes revealed that they play a critical part in the parasites’ development more specially at the liver stage. Thus, providing new and renewed insight and interests in developing live attenuated vaccine against malaria (129,130). The UIS3 gene is one of the genetic factors that is implicated in the synthesis of type I transmembrane proteins. It can be inserted into the membrane of infected hepatocytes and exposed to the cytoplasm. A two-hybrid screen revealed that the presence of this protein in the exposed domain interacted with a protein that binds to liver fatty acids (131). Mikolajczak et al., demonstrated that the L-FABP plays a crucial role as host factor for effective malaria liver stage growth and that the communication between the UIS3 and L-FABP is vital for the parasite’s development. Based on literature, it is believed that the presence of the latter in the host cell promotes the uptake of fatty acid. The recruitment of L-FABP into the PV membrane could allow the parasites to transport more fatty acids (131).
6.1. Mechanisms of Apicomplexans using Alternative Routes for Fatty Acid Synthesis
As described in Mazumdar & Striepen (111), T. gondii incorporation of acetate into fatty acids does not rely on FASII pathways. In Apicomplexan Cryptosporidium parvum, there is an alternative pathway described, as it does not also rely on the FASII pathway because Apicomplexan Cryptosporidium parvum lacks it as well as the apicoplast organelle. However Apicomplexan Cryptosporidium parvum genome encodes a large (~900-kDa) FASII-like enzymes, which contains four ACP domains (loading unit, putative terminating reductase domain, reduction, and three elongation modules) (14). Phylogenetic studies have likened this enzyme to that of the bacteria polyketide synthase (PKSs). The large size of the FASI gene makes it difficult to study it (132). CoA is an alternative way of measuring the enzyme activities (6). Additionally, putative PKS is the second related gene encoded by C. parvum, and this protein uses palmitoyl-CoA substrate and comprises seven acyl elongation units of different compositions. It also has unknown end products catalyzed by CpPKS. Both bacteria and fungi are known to synthesize PKS and they are used for a number of purposes including their uses as siderophores, toxins, and antibiotics (133,134). Dinoflagellates are the only known phylum of the Apicomplexan groups known to synthesize multitudes of PK toxins. Karen brevis is known to be the first from which dinoflagellate genes were identified and was similar to CpPKS (135). Similar genes shared by CpPKS and dinoflagellates suggest a horizontal gene transfer between the two before their division. Three huge FASI/PKStype genes are contained within the genome of T. gondii, and one of the genes (ToxoDB gene ID 83.m00010) encodes FASI, and the remaining two genes possibly encode for PKSs. FASI and PKS may play a significant role in the life cycle stages of Cryptosporidium, Eimeria, and Toxoplasma, as a constituent of the impermeable and resilient oocyst wall (12).
The fatty acid elongation system is the third method of synthesizing fatty acids, and the enzymatic procedures are the same as in FAS I & II. However, CoA and not ACP support the growing chain. Fatty acid elongation is established in the endoplasmic reticulum and aids in the sequential elongation of fatty acids, thus providing the needed long-chain fatty acids during the production of phospholipids (136). Elongations 1 & 2 are a critical source of myristic acid for the glycosylphosphatidylinositol held firmly the highly abundant variable surface glycoprotein of the bloodstream stage, as suggested by genomic data (137). Three elongated genes were recognized in P. falciparum as well as in T. gondii, and one was identified in Cryptosporidium. These genes serve as another source of FASII-independent 14C acetate incorporation in T. gondii. This is on basis that these genes are implicated in the production of longer fatty acids and possesses high sensitivity to cerulenin (138). Apicomplexans elongations fatty acids may be engaged in the standard elongation process instead of de novo synthesis. The synthesis of fatty acid relies on acetyl-CoA, malonyl-CoA, energy, and reduction potential of ATP and NADH(P)H. Cytoplasmic glycolysis is crucial in the supply of phosphoenolpyruvate, which is subsequently transported to the plasmid and later metabolized to acetyl-CoA (139). P. falciparum has two identified putative apicoplast phosphor-sugar transporters with one component constituting the outermost and the other constituting the innermost membrane, though four membranes bound the apicoplast (140). Some observational studies have suggested that apicoplasts likely import phosphoenolpyruvate. Hence apicoplast has pyruvate and phosphoenolpyruvate, including dihydroxyacetone phosphate. Aside from the provision of acetyl-CoA, PDH, and pyruvate kinase also generates ATP and NAD(P)H, which shuttles the reducing agents into the organelles (141).
References
- Sibley, L.D. How apicomplexan parasites move in and out of cells. Curr. Opin. Biotechnol. 2010, 21, 592–598. [Google Scholar] [CrossRef] [PubMed]
- Huffman, A.M.; Ayariga, J.A.; Napier, A.; Robertson, B.K.; Abugri, D.A. Inhibition of Toxoplasma gondii Growth by Dihydroquinine and Its Mechanisms of Action. Front. Cell. Infect. Microbiol. 2022, 12, 852889. [Google Scholar] [CrossRef] [PubMed]
- Blackman, M.J.; Bannister, L.H. Apical organelles of Apicomplexa: biology and isolation by subcellular fractionation. Mol. Biochem. Parasitol. 2001, 117, 11–25. [Google Scholar] [CrossRef] [PubMed]
- Cruz-Bustos, T.; Feix, A.S.; Ruttkowski, B.; Joachim, A. Sexual development in non-human parasitic Apicomplexa: just biology or targets for control? Animals 2021, 11, 2891. [Google Scholar] [CrossRef] [PubMed]
- Abugri, J.; Ayariga, J.; Sunwiale, S.S.; Wezena, C.A.; Gyamfi, J.A.; Adu-Frimpong, M.; Agongo, G.; Dongdem, J.T.; Abugri, D.; Dinko, B. Targeting the Plasmodium falciparum proteome and organelles for potential antimalarial drug candidates. Heliyon 2022, 8, e10390. [Google Scholar] [CrossRef] [PubMed]
- Ramakrishnan, S.; Serricchio, M.; Striepen, B.; Bütikofer, P. Lipid synthesis in protozoan parasites: A comparison between kinetoplastids and apicomplexans. Prog. Lipid Res. 2013, 52, 488–512. [Google Scholar] [CrossRef] [PubMed]
- Coppens, I. Targeting lipid biosynthesis and salvage in apicomplexan parasites for improved chemotherapies. Nat. Rev. Microbiol. 2013, 11, 823–835. [Google Scholar] [CrossRef] [PubMed]
- Lell, B.; Ruangweerayut, R.; Wiesner, J.; Missinou, M.A.; Schindler, A.; Baranek, T.; Hintz, M.; Hutchinson, D.; Jomaa, H.; Kremsner, P.G. Fosmidomycin, a Novel Chemotherapeutic Agent for Malaria. Antimicrob. Agents Chemother. 2003, 47, 735–738. [Google Scholar] [CrossRef]
- World Health Organization. Accelerating progress on HIV, tuberculosis, malaria, hepatitis and neglected tropical diseases: A new agenda for 2016–2030. 2015. [Google Scholar]
- Awoke, N.; Arota, A. Profiles of hematological parameters in Plasmodium falciparum and Plasmodium vivax malaria patients attending Tercha General Hospital, Dawuro Zone, South Ethiopia. Infect. Drug Resist. 2019, ume 12, 521–527. [Google Scholar] [CrossRef]
- Smith, T.G.; Walliker, D.; Ranford-Cartwright, L.C. Sexual differentiation and sex determination in the Apicomplexa. Trends Parasitol. 2002, 18, 315–323. [Google Scholar] [CrossRef]
- Zhu, G.; LaGier, M.J.; Stejskal, F.; Millership, J.J.; Cai, X.; Keithly, J.S. Cryptosporidium parvum: the first protist known to encode a putative polyketide synthase. Gene 2002, 298, 79–89. [Google Scholar] [CrossRef] [PubMed]
- Zhu, G.; Marchewka, M.J.; Woods, K.M.; Upton, S.J.; Keithly, J.S. Molecular analysis of a Type I fatty acid synthase in Cryptosporidium parvum. Mol. Biochem. Parasitol. 2000, 105, 253–260. [Google Scholar] [CrossRef] [PubMed]
- Zhu, G.; Li, Y.; Cai, X.; Millership, J.J.; Marchewka, M.J.; Keithly, J.S. Expression and functional characterization of a giant Type I fatty acid synthase (CpFAS1) gene from Cryptosporidium parvum. Mol. Biochem. Parasitol. 2003, 134, 127–135. [Google Scholar] [CrossRef] [PubMed]
- Chintu, C.; Luo, C.; Bhat, G.; DuPont, H.L.; Mwansa-Salamu, P.; Kabika, M.; Zumla, A. Impact of the Human Immunodeficiency Virus Type-1 on Common Pediatric Illnesses in Zambia. J. Trop. Pediatr. 1995, 41, 348–353. [Google Scholar] [CrossRef] [PubMed]
- Ryan, U.; Zahedi, A.; Paparini, A. Cryptosporidium in humans and animals—a one health approach to prophylaxis. Parasite immunology 2016, 38, 535–547. [Google Scholar] [CrossRef] [PubMed]
- Sponseller, J.K.; Griffiths, J.K.; Tzipori, S. The Evolution of Respiratory Cryptosporidiosis: Evidence for Transmission by Inhalation. Clin. Microbiol. Rev. 2014, 27, 575–586. [Google Scholar] [CrossRef] [PubMed]
- Maikai, B.V.; Baba-Onoja, E.B.T.; Elisha, I.A. Contamination of raw vegetables with Cryptosporidium oocysts in markets within Zaria metropolis, Kaduna State, Nigeria. Food Control 2013, 31, 45–48. [Google Scholar] [CrossRef]
- Shanmugam, D.; Wu, B.; Ramirez, U.; Jaffe, E.K.; Roos, D.S. Plastid-associated porphobilinogen synthase from Toxoplasma gondii: kinetic and structural properties validate therapeutic potential. J. Biol. Chem. 2010, 285, 22122–22131. [Google Scholar] [CrossRef] [PubMed]
- Divo, A.A.; Geary, T.G.; Jensen, J.B.; Ginsburg, H. The Mitochondrion of Plasmodium falciparum Visualized by Rhodamine 123 Fluorescence 1. J. Protozool. 1985, 32, 442–446. [Google Scholar] [CrossRef]
- Fayer, R. General biology. In Cryptosporidium and cryptosporidiosis; CRC press: Boca Raton, FL, USA, 2007; pp. 1–42. [Google Scholar]
- Billker, O.; Lourido, S.; Sibley, L.D. Calcium-Dependent Signaling and Kinases in Apicomplexan Parasites. Cell Host Microbe 2009, 5, 612–622. [Google Scholar] [CrossRef]
- Nagamune, K.; Moreno, S.N.; Chini, E.N.; Sibley, L.D. Calcium regulation and signaling in Apicomplexan parasites. Mol. Mech. Parasite Invasion 2008, 70–81. [Google Scholar]
- Nagamune, K.; Beatty, W.L.; Sibley, L.D. Artemisinin Induces Calcium-Dependent Protein Secretion in the Protozoan ParasiteToxoplasma gondii. Eukaryot. Cell 2007, 6, 2147–2156. [Google Scholar] [CrossRef] [PubMed]
- Ghartey-Kwansah, G.; Yin, Q.; Li, Z.; Gumpper, K.; Sun, Y.; Yang, R.; Wang, D.; Jones, O.; Zhou, X.; Wang, L.; et al. Calcium-dependent Protein Kinases in Malaria Parasite Development and Infection. Cell Transplant. 2020, 29. [Google Scholar] [CrossRef]
- Nagamune, K.; Sibley, L.D. Comparative Genomic and Phylogenetic Analyses of Calcium ATPases and Calcium-Regulated Proteins in the Apicomplexa. Mol. Biol. Evol. 2006, 23, 1613–1627. [Google Scholar] [CrossRef] [PubMed]
- Cavalcanti, J.H.F.; Esteves-Ferreira, A.A.; Quinhones, C.G.; Pereira-Lima, I.A.; Nunes-Nesi, A.; Fernie, A.R.; Araújo, W.L. Evolution and Functional Implications of the Tricarboxylic Acid Cycle as Revealed by Phylogenetic Analysis. Genome Biol. Evol. 2014, 6, 2830–2848. [Google Scholar] [CrossRef] [PubMed]
- Stammers, A.N.; Susser, S.E.; Hamm, N.C.; Hlynsky, M.W.; Kimber, D.E.; Kehler, D.S.; Duhamel, T.A. The regulation of sarco(endo)plasmic reticulum calcium-ATPases (SERCA). Can. J. Physiol. Pharmacol. 2015, 93, 843–854. [Google Scholar] [CrossRef] [PubMed]
- Mogi, T.; Kita, K. Diversity in mitochondrial metabolic pathways in parasitic protists Plasmodium and Cryptosporidium. Parasitol. Int. 2010, 59, 305–312. [Google Scholar] [CrossRef] [PubMed]
- Lambalot, R.H.; Walsh, C.T. Cloning, Overproduction, and Characterization of the Escherichia coli Holo-acyl Carrier Protein Synthase (∗). J. Biol. Chem. 1995, 270, 24658–24661. [Google Scholar] [CrossRef]
- Coombs, G.; Müller, S. Recent advances in the search for new anti-coccidial drugs. Int. J. Parasitol. 2002, 32, 497–508. [Google Scholar] [CrossRef]
- Fry, M.; Beesley, J.E. Mitochondria of mammalian Plasmodium spp. Parasitology 1991, 102, 17–26. [Google Scholar] [CrossRef]
- Sorrentino, V.; Barone, V.; Rossi, D. Intracellular Ca2+ release channels in evolution. Curr. Opin. Genet. Dev. 2000, 10, 662–667. [Google Scholar] [CrossRef] [PubMed]
- Hopkins, J.; Fowler, R.; Krishna, S.; Wilson, I.; Mitchell, G.; Bannister, L. The Plastid in Plasmodium falciparum Asexual Blood Stages: a Three-Dimensional Ultrastructural Analysis. Protist 1999, 150, 283–295. [Google Scholar] [CrossRef]
- Williams, B.A.P.; Hirt, R.P.; Lucocq, J.M.; Embley, T.M. A mitochondrial remnant in the microsporidian Trachipleistophora hominis. Nature 2002, 418, 865–869. [Google Scholar] [CrossRef] [PubMed]
- McIntosh, L. Molecular Biology of the Alternative Oxidase. Plant Physiol. 1994, 105, 781–786. [Google Scholar] [CrossRef]
- Cavalier-Smith, T. The phagotrophic origin of eukaryotes and phylogenetic classification of Protozoa. Int. J. Syst. Evol. Microbiol. 2002, 52, 297–354. [Google Scholar] [CrossRef] [PubMed]
- Lambalot, R.H.; Gehring, A.M.; Flugel, R.S.; Zuber, P.; LaCelle, M.; Marahiel, M.A.; Reid, R.; Khosla, C.; Walsh, C.T. A new enzyme superfamily—The phosphopantetheinyl transferases. Chem. Biol. 1996, 3, 923–936. [Google Scholar] [CrossRef]
- Gornicki, P. Apicoplast fatty acid biosynthesis as a target for medical intervention in apicomplexan parasites. Int. J. Parasitol. 2003, 33, 885–896. [Google Scholar] [CrossRef]
- Rotte, C.; Stejskal, F.; Zhu, G.; Keithly, J.S.; Martin, W. Pyruvate:NADP Oxidoreductase from the Mitochondrion of Euglena gracilis and from the Apicomplexan Cryptosporidium parvum: A Biochemical Relic Linking Pyruvate Metabolism in Mitochondriate and Amitochondriate Protists. Mol. Biol. Evol. 2001, 18, 710–720. [Google Scholar] [CrossRef] [PubMed]
- Spork, S.; Hiss, J.A.; Mandel, K.; Sommer, M.; Kooij, T.W.A.; Chu, T.; Schneider, G.; Maier, U.G.; Przyborski, J.M. An Unusual ERAD-Like Complex Is Targeted to the Apicoplast ofPlasmodium falciparum. Eukaryot. Cell 2009, 8, 1134–1145. [Google Scholar] [CrossRef]
- Taraschi, T.F.; Trelka, D.; Martinez, S.; Schneider, T.; E O'Donnell, M. Vesicle-mediated trafficking of parasite proteins to the host cell cytosol and erythrocyte surface membrane in Plasmodium falciparum infected erythrocytes. Int. J. Parasitol. 2001, 31, 1381–1391. [Google Scholar] [CrossRef]
- Tsien, R.W.; Tsien, R.Y. Calcium channels, stores, and oscillations. Annu. Rev. Cell Biol. 1990, 6, 715–760. [Google Scholar] [CrossRef] [PubMed]
- Uni, S.; Iseki, M.; Maekawa, T.; Moriya, K.; Takada, S. Ultrastructure of Cryptosporidium muris (strain RN 66) parasitizing the murine stomach. Parasitol. Res. 1987, 74, 123–132. [Google Scholar] [CrossRef] [PubMed]
- Vanlerberghe, G.C.; McIntosh, L. ALTERNATIVE OXIDASE: From Gene to Function. Annu. Rev. Plant Physiol. Plant Mol. Biol. 1997, 48, 703–734. [Google Scholar] [CrossRef] [PubMed]
- Miller, R.J.; Fox, A.P. Voltage sensitive calcium channels. In Intracellular calcium regulation; Bronner, F., Ed.; Wiley-Liss, 1990; pp. 97–138. [Google Scholar]
- Kalanon, M.; Tonkin, C.J.; McFadden, G.I. Characterization of Two Putative Protein Translocation Components in the Apicoplast ofPlasmodium falciparum. Eukaryot. Cell 2009, 8, 1146–1154. [Google Scholar] [CrossRef]
- Mootz, H.D.; Finking, R.; Marahiel, M.A. 4′-Phosphopantetheine Transfer in Primary and Secondary Metabolism of Bacillus subtilis. J. Biol. Chem. 2001, 276, 37289–37298. [Google Scholar] [CrossRef]
- Philippe, H.; Lopez, P.; Brinkmann, H.; Budin, K.; Germot, A.; Laurent, J.; Moreira, D.; Müller, M.; Guyader, H.L. Early–branching or fast–evolving eukaryotes? An answer based on slowly evolving positions. Proc. R. Soc. Lond. Ser. B Biol. Sci. 2000, 267, 1213–1221. [Google Scholar] [CrossRef]
- Plattner, H. My favorite cell—Paramecium. Bioessays 2002, 24, 649–658. [Google Scholar] [CrossRef]
- Ponting, C.; Schultz, J.; Bork, P. SPRY domains in ryanodine receptors (Ca2+-release channels). Trends Biochem. Sci. 1997, 22, 193–194. [Google Scholar] [CrossRef]
- Praphanphoj, V.; Sacksteder, K.A.; Gould, S.J.; Thomas, G.H.; Geraghty, M.T. Identification of the α-aminoadipic semialdehyde dehydrogenase-phosphopantetheinyl transferase gene, the human ortholog of the yeast LYS5 gene. Mol. Genet. Metab. 2001, 72, 336–342. [Google Scholar] [CrossRef]
- Neal, A.T.; Jordan, S.J.; Oliveira, A.L.; Hernandez, J.N.; Branch, O.H.; Rayner, J.C. Limited variation in vaccine candidate Plasmodium falciparum Merozoite Surface Protein-6 over multiple transmission seasons. Malar. J. 2010, 9, 138–138. [Google Scholar] [CrossRef]
- Brown, S.M.; McDonald, V.; Denton, H.; Coombs, G.H. The use of a new viability assay to determine the susceptibility of Cryptosporidium and Eimeria sporozoites to respiratory inhibitors and extremes of pH. FEMS Microbiol. Lett. 1996, 142, 203–208. [Google Scholar] [CrossRef] [PubMed]
- Quadri, L.E.; Weinreb, P.H.; Lei, M.; Nakano, M.M.; Zuber, P.; Walsh, C.T. Characterization of Sfp, a Bacillus subtilis phosphopantetheinyl transferase for peptidyl carrier protein domains in peptide synthetases. Biochemistry 1998, 37, 1585–1595. [Google Scholar] [CrossRef] [PubMed]
- Wetzel, C.M.; Jiang, C.; Meehan, L.J.; Voytas, D.F.; Rodermel, S.R. Nuclear—organelle interactions: the immutans variegation mutant of Arabidopsis is plastid autonomous and impaired in carotenoid biosynthesis. Plant J. 1994, 6, 161–175. [Google Scholar] [CrossRef]
- Foth, B.J.; Ralph, S.A.; Tonkin, C.J.; Struck, N.S.; Fraunholz, M.; Roos, D.S.; Cowman, A.F.; McFadden, G.I. Dissecting Apicoplast Targeting in the Malaria Parasite Plasmodium falciparum. Science 2003, 299, 705–708. [Google Scholar] [CrossRef] [PubMed]
- Martin, W.; Russell, M.J. On the origins of cells: a hypothesis for the evolutionary transitions from abiotic geochemistry to chemoautotrophic prokaryotes, and from prokaryotes to nucleated cells. Philos. Trans. R. Soc. B Biol. Sci. 2003, 358, 59–85. [Google Scholar] [CrossRef] [PubMed]
- Joshi, A.K.; Zhang, L.; Rangan, V.S.; Smith, S. Cloning, expression, and characterization of a human 4′-phosphopantetheinyl transferase with broad substrate specificity. J. Biol. Chem. 2003, 278, 33142–33149. [Google Scholar] [CrossRef] [PubMed]
- Arnou, B.; Montigny, C.; Morth, J.P.; Nissen, P.; Jaxel, C.; Møller, J.V.; Maire, M.L. The Plasmodium falciparum Ca2+-ATPase PfATP6: insensitive to artemisinin, but a potential drug target. 2011. [Google Scholar]
- Madern, D.; Ebel, C.; Mevarech, M.; Richard, S.B.; Pfister, C.; Zaccai, G. Insights into the Molecular Relationships between Malate and Lactate Dehydrogenases: Structural and Biochemical Properties of Monomeric and Dimeric Intermediates of a Mutant of Tetrameric l-[LDH-like] Malate Dehydrogenase from the Halophilic Archaeon Haloarcula marismortui. Biochemistry 2000, 39, 1001–1010. [Google Scholar] [CrossRef] [PubMed]
- Gardner, M.J.; Hall, N.; Fung, E.; White, O.; Berriman, M.; Hyman, R.W.; Carlton, J.M.; Pain, A.; Nelson, K.E.; Bowman, S.; et al. Genome sequence of the human malaria parasite Plasmodium falciparum. Nature 2002, 419, 498–511. [Google Scholar] [CrossRef]
- A Travassos, M.; Laufer, M.K. Resistance to Antimalarial Drugs: Molecular, Pharmacologic, and Clinical Considerations. Pediatr. Res. 2009, 65, 64R–70R. [Google Scholar] [CrossRef] [PubMed]
- Ferreira, P. Molecular basis for the mechanism of action and resistance to artemisinin combination therapy in Plasmodium falciparum. Inst för medicin, Solna/Dept of Medicine, Solna. 2010. [Google Scholar]
- Fisher, N.; Bray, P.G.; Ward, S.A.; Biagini, G.A. The malaria parasite type II NADH:quinone oxidoreductase: an alternative enzyme for an alternative lifestyle. Trends Parasitol. 2007, 23, 305–310. [Google Scholar] [CrossRef]
- Krungkrai, J. The multiple roles of the mitochondrion of the malarial parasite. Parasitology 2004, 129, 511–524. [Google Scholar] [CrossRef] [PubMed]
- Ehrhardt, K.; Davioud-Charvet, E.; Ke, H.; Vaidya, A.B.; Lanzer, M.; Deponte, M. The antimalarial activities of methylene blue and the 1, 4-naphthoquinone 3-[4-(trifluoromethyl) benzyl]-menadione are not due to inhibition of the mitochondrial electron transport chain. Antimicrob. Agents Chemother. 2013, 57, 2114–2120. [Google Scholar] [CrossRef] [PubMed]
- Fleige, T.; Pfaff, N.; Gross, U.; Bohne, W. Localisation of gluconeogenesis and tricarboxylic acid (TCA)-cycle enzymes and first functional analysis of the TCA cycle in Toxoplasma gondii. Int. J. Parasitol. 2008, 38, 1121–1132. [Google Scholar] [CrossRef] [PubMed]
- Germot, A.; Philippe, H.; Le Guyader, H. Presence of a mitochondrial-type 70-kDa heat shock protein in Trichomonas vaginalis suggests a very early mitochondrial endosymbiosis in eukaryotes. Proc. Natl. Acad. Sci. 1996, 93, 14614–14617. [Google Scholar] [CrossRef] [PubMed]
- Roberts, C.W.; Roberts, F.; Henriquez, F.L.; Akiyoshi, D.; Samuel, B.U.; A Richards, T.; Milhous, W.; Kyle, D.; McIntosh, L.; Hill, G.C.; et al. Evidence for mitochondrial-derived alternative oxidase in the apicomplexan parasite Cryptosporidium parvum: a potential anti-microbial agent target. Int. J. Parasitol. 2004, 34, 297–308. [Google Scholar] [CrossRef]
- Miranda, K.; Benchimol, M.; Docampo, R.; de Souza, W. The fine structure of acidocalcisomes in Trypanosoma cruzi. Parasitol. Res. 2000, 86, 373–384. [Google Scholar] [CrossRef] [PubMed]
- Rizzuto, R.; Brini, M.; Murgia, M.; Pozzan, T. Microdomains with high Ca2+ close to IP3-sensitive channels that are sensed by neighboring mitochondria. Science 1993, 262, 744–747. [Google Scholar] [CrossRef] [PubMed]
- Huang, G.; Bartlett, P.J.; Thomas, A.P.; Moreno, S.N.J.; Docampo, R. Acidocalcisomes of Trypanosoma brucei have an inositol 1,4,5-trisphosphate receptor that is required for growth and infectivity. Proc. Natl. Acad. Sci. 2013, 110, 1887–1892. [Google Scholar] [CrossRef] [PubMed]
- Xiong, Z.H.; Ridgley, E.L.; Enis, D.; Olness, F.; Ruben, L. Selective Transfer of Calcium from an Acidic Compartment to the Mitochondrion of Trypanosoma brucei: MEASUREMENTS WITH TARGETED AEQUORINS. J. Biol. Chem. 1997, 272, 31022–31028. [Google Scholar] [CrossRef]
- Docampo, R. The origin and evolution of the acidocalcisome and its interactions with other organelles. Mol. Biochem. Parasitol. 2015, 209, 3–9. [Google Scholar] [CrossRef]
- Baker, N.; Hamilton, G.; Wilkes, J.M.; Hutchinson, S.; Barrett, M.P.; Horn, D. Vacuolar ATPase depletion affects mitochondrial ATPase function, kinetoplast dependency, and drug sensitivity in trypanosomes. Proc. Natl. Acad. Sci. 2015, 112, 9112–9117. [Google Scholar] [CrossRef] [PubMed]
- Huang, G.; Ulrich, P.N.; Storey, M.; Johnson, D.; Tischer, J.; Tovar, J.A.; Moreno, S.N.J.; Orlando, R.; Docampo, R. Proteomic Analysis of the Acidocalcisome, an Organelle Conserved from Bacteria to Human Cells. PLOS Pathog. 2014, 10, e1004555. [Google Scholar] [CrossRef]
- Rohloff, P.; Rodrigues, C.O.; Docampo, R. Regulatory volume decrease in Trypanosoma cruzi involves amino acid efflux and changes in intracellular calcium. Mol. Biochem. Parasitol. 2002, 126, 219–230. [Google Scholar] [CrossRef]
- Benaim, G.; Paniz-Mondolfi, A.E.; Sordillo, E.M.; Martinez-Sotillo, N. Disruption of Intracellular Calcium Homeostasis as a Therapeutic Target Against Trypanosoma cruzi. Front. Cell. Infect. Microbiol. 2020, 10, 46. [Google Scholar] [CrossRef]
- Docampo, R.; A Scott, D.; E Vercesi, A.; Moreno, S.N.J. Intracellular Ca2+ storage in acidocalcisomes of Trypanosoma cruzi. Biochem. J. 1995, 310, 1005–1012. [Google Scholar] [CrossRef]
- Mendoza, M.; Mijares, A.; Rojas, H.; Rodrı́guez, J.; Urbina, J.; DiPolo, R. Physiological and morphological evidences for the presence acidocalcisomes in Trypanosoma evansi: Single cell fluorescence and 31P NMR studies. Mol. Biochem. Parasitol. 2002, 125, 23–33. [Google Scholar] [CrossRef]
- Moreno-Sanchez, D.; Hernandez-Ruiz, L.; Ruiz, F.A.; Docampo, R. Polyphosphate Is a Novel Pro-inflammatory Regulator of Mast Cells and Is Located in Acidocalcisomes. J. Biol. Chem. 2012, 287, 28435–28444. [Google Scholar] [CrossRef] [PubMed]
- Souza, W. An Introduction to the Structural Organization of Parasitic Protozoa. Curr. Pharm. Des. 2008, 14, 822–838. [Google Scholar] [CrossRef] [PubMed]
- Jimenez, V.; Mesones, S. Down the membrane hole: Ion channels in protozoan parasites. PLOS Pathog. 2022, 18, e1011004. [Google Scholar] [CrossRef]
- Johansson, N. Design and synthesis of membrane-bound pyrophosphatase inhibitors against pathogenic protozoan parasites. Doctoral School in Natural Sciences Dissertation Series. 2022.
- Rodrigues, C.O.; Scott, D.A.; Docampo, R. Presence of a vacuolar H+-pyrophosphatase in promastigotes of Leishmania donovani and its localization to a different compartment from the vacuolar H+-ATPase. Biochem. J. 1999, 340, 759–766. [Google Scholar] [CrossRef] [PubMed]
- Docampo, R.; de Souza, W.; Miranda, K.; Rohloff, P.; Moreno, S.N.J. Acidocalcisomes ? conserved from bacteria to man. Nat. Rev. Microbiol. 2005, 3, 251–261. [Google Scholar] [CrossRef]
- Biagini, G.A.; Bray, P.G.; Spiller, D.G.; White, M.R.H.; Ward, S.A. The Digestive Food Vacuole of the Malaria Parasite Is a Dynamic Intracellular Ca2+ Store. J. Biol. Chem. 2003, 278, 27910–27915. [Google Scholar] [CrossRef] [PubMed]
- Souza, W.D.; Carreiro, I.P.; Miranda, K.; SILVA, N.L.C.E. Two special organelles found in Trypanosoma cruzi. An. Acad. Bras. Ciências 2000, 72, 421–432. [Google Scholar] [CrossRef]
- Ruben, L.; Kelly, J.M.; Chakrabarti, D. Intracellular signaling. In Molecular medical parasitology; Academic Press, 2003; pp. 241–VIII. [Google Scholar]
- Vercesi, E.A.; Grijalba, T.M.; Docampo, R. Inhibition of Ca2+ release from Trypanosoma brucei acidocalcisomes by 3,5-dibutyl-4-hydroxytoluene: role of the Na+/H+ exchanger. Biochem. J. 1997, 328, 479–482. [Google Scholar] [CrossRef] [PubMed]
- Vercesi, A.E.; Rodrigues, C.O.; Catisti, R.; Docampo, R. Presence of a Na+/H+ exchanger in acidocalcisomes of Leishmania donovani and their alkalization by anti-leishmanial drugs. FEBS Lett. 2000, 473, 203–206. [Google Scholar] [CrossRef] [PubMed]
- Raley-Susman, K.M.; Cragoe, E.J., Jr.; Sapolsky, R.M.; Kopito, R.R. Regulation of intracellular pH in cultured hippocampal neurons by an amiloride-insensitive Na+/H+ exchanger. J. Biol. Chem. 1991, 266, 2739–2745. [Google Scholar] [CrossRef] [PubMed]
- Wunderlich, J.; Rohrbach, P.; Dalton, J.P. The malaria digestive vacuole. Front. Biosci. -Sch. 2012, 4, 1424–1448. [Google Scholar]
- Bailey, B.N. Bisphosphonates and aryloxyethyl thiocyanate derivatives: New drugs against trypanosomatid parasites; University of Illinois at Urbana-Champaign. 2004. [Google Scholar]
- Wunderlich, J. The effect of antimalarial drugs on intracellular pH in Plasmodium falciparum; McGill University (Canada), 2018. [Google Scholar]
- King-Keller, S.; Moore, C.A.; Docampo, R.; Moreno, S.N.J. Ca2+ Regulation of Trypanosoma brucei Phosphoinositide Phospholipase C. Eukaryot. Cell 2015, 14, 486–494. [Google Scholar] [CrossRef]
- Keller, S.K. Investigations of signaling pathways and their potential benefits in combating trypanosomatid diseases. Doctoral dissertation, University of Georgia, 2013. [Google Scholar]
- Moreno, B.; Urbina, J.A.; Oldfield, E.; Bailey, B.N.; Rodrigues, C.O.; Docampo, R. 31P NMR spectroscopy of Trypanosoma brucei, Trypanosoma cruzi, and Leishmania major: Evidence for high levels of condensed inorganic phosphates. J. Biol. Chem. 2000, 275, 28356–28362. [Google Scholar] [CrossRef]
- Docampo, R.; Moreno, S.N. The acidocalcisome. Molecular and biochemical parasitology 2001, 114, 151–159. [Google Scholar] [CrossRef]
- Ruiz, F.A.; Rodrigues, C.O.; Docampo, R. Rapid Changes in Polyphosphate Content within Acidocalcisomes in Response to Cell Growth, Differentiation, and Environmental Stress inTrypanosoma cruzi. J. Biol. Chem. 2001, 276, 26114–26121. [Google Scholar] [CrossRef]
- Jimenez, V. Dealing with environmental challenges: Mechanisms of adaptation in Trypanosoma cruzi. Res. Microbiol. 2014, 165, 155–165. [Google Scholar] [CrossRef]
- Moreno, B.; Bailey, B.N.; Luo, S.; Martin, M.B.; Kuhlenschmidt, M.; Moreno, S.N.; Docampo, R.; Oldfield, E. 31P NMR of Apicomplexans and the Effects of Risedronate on Cryptosporidium parvum Growth. Biochem. Biophys. Res. Commun. 2001, 284, 632–637. [Google Scholar] [CrossRef]
- Jordão, F.M.; Saito, A.Y.; Miguel, D.C.; de Jesus Peres, V.; Kimura, E.A.; Katzin, A.M. In vitro and in vivo antiplasmodial activities of risedronate and its interference with protein prenylation in Plasmodium falciparum. Antimicrob. Agents Chemother. 2011, 55, 2026–2031. [Google Scholar] [CrossRef]
- Rodrigues, C.O.; Scott, D.A.; Bailey, B.N.; DE Souza, W.; Benchimol, M.; Moreno, B.; Urbina, J.A.; Oldfield, E.; Moreno, S.N.J. Vacuolar proton pyrophosphatase activity and pyrophosphate (PPi) in Toxoplasma gondii as possible chemotherapeutic targets. Biochem. J. 2000, 349, 737–745. [Google Scholar] [CrossRef]
- Luo, S.; Marchesini, N.; Moreno, S.N.; Docampo, R. A plant-like vacuolar H+-pyrophosphatase in Plasmodium falciparum. FEBS Lett. 1999, 460, 217–220. [Google Scholar] [CrossRef]
- Docampo, R.; Moreno, S.N. The Acidocalcisome as a Target for Chemotherapeutic Agents in Protozoan Parasites. Curr. Pharm. Des. 2008, 14, 882–888. [Google Scholar] [CrossRef]
- Westermann, B.; Neupert, W. Mitochondria-targeted green fluorescent proteins: convenient tools for the study of organelle biogenesis in Saccharomyces cerevisiae. Yeast 2000, 16, 1421–1427. [Google Scholar] [CrossRef]
- Wuytack, F.; Raeymaekers, L.; Missiaen, L. Molecular physiology of the SERCA and SPCA pumps. Cell Calcium 2002, 32, 279–305. [Google Scholar] [CrossRef] [PubMed]
- Zhu, G. Current Progress in the Fatty Acid Metabolism in Cryptosporidium parvum 1. J. Eukaryot. Microbiol. 2004, 51, 381–388. [Google Scholar] [CrossRef] [PubMed]
- Mazumdar, J.; Striepen, B. Make It or Take It: Fatty Acid Metabolism of Apicomplexan Parasites. Eukaryot. Cell 2007, 6, 1727–1735. [Google Scholar] [CrossRef]
- Gerold, P.; Dieckmann-Schuppert, A.; Schwarz, R. Glycosylphosphatidylinositols synthesized by asexual erythrocytic stages of the malarial parasite, Plasmodium falciparum. Candidates for plasmodial glycosylphosphatidylinositol membrane anchor precursors and pathogenicity factors. J. Biol. Chem. 1994, 269, 2597–2606. [Google Scholar] [CrossRef] [PubMed]
- Tomavo, S.; Dubremetz, J.; Schwarz, R. A family of glycolipids from Toxoplasma gondii. Identification of candidate glycolipid precursor(s) for Toxoplasma gondii glycosylphosphatidylinositol membrane anchors. J. Biol. Chem. 1992, 267, 11721–11728. [Google Scholar] [CrossRef] [PubMed]
- Charron, A.J.; Sibley, L.D. Host cells: mobilizable lipid resources for the intracellular parasite Toxoplasma gondii. J. Cell Sci. 2002, 115, 3049–3059. [Google Scholar] [CrossRef] [PubMed]
- Vielemeyer, O.; McIntosh, M.T.; Joiner, K.A.; Coppens, I. Neutral lipid synthesis and storage in the intraerythrocytic stages of Plasmodium falciparum. Mol. Biochem. Parasitol. 2004, 135, 197–209. [Google Scholar] [CrossRef] [PubMed]
- Quittnat, F.; Nishikawa, Y.; Stedman, T.T.; Voelker, D.R.; Choi, J.-Y.; Zahn, M.M.; Murphy, R.C.; Barkley, R.M.; Pypaert, M.; Joiner, K.A.; et al. On the biogenesis of lipid bodies in ancient eukaryotes: synthesis of triacylglycerols by a Toxoplasma DGAT1-related enzyme. Mol. Biochem. Parasitol. 2004, 138, 107–122. [Google Scholar] [CrossRef] [PubMed]
- Bethke, L.L.; Zilversmit, M.; Nielsen, K.; Daily, J.; Volkman, S.K.; Ndiaye, D.; Lozovsky, E.R.; Hartl, D.L.; Wirth, D.F. Duplication, gene conversion, and genetic diversity in the species-specific acyl-CoA synthetase gene family of Plasmodium falciparum. Mol. Biochem. Parasitol. 2006, 150, 10–24. [Google Scholar] [CrossRef] [PubMed]
- Zeng, B.; Cai, X.; Zhu, G. Functional characterization of a fatty acyl-CoA-binding protein (ACBP) from the Apicomplexan Cryptosporidium parvum. Microbiology 2006, 152, 2355–2363. [Google Scholar] [CrossRef]
- Zeng, B.; Zhu, G. Two distinct oxysterol binding protein-related proteins in the parasitic protist Cryptosporidium parvum (Apicomplexa). Biochem. Biophys. Res. Commun. 2006, 346, 591–599. [Google Scholar] [CrossRef]
- Sinai, A.P.; Webster, P.; Joiner, K.A. Association of host cell endoplasmic reticulum and mitochondria with the Toxoplasma gondii parasitophorous vacuole membrane: A high affinity interaction. J. Cell Sci. 1997, 110, 2117–2128. [Google Scholar] [CrossRef] [PubMed]
- Bano, N.; Romano, J.D.; Jayabalasingham, B.; Coppens, I. Cellular interactions of Plasmodium liver stage with its host mammalian cell. Int. J. Parasitol. 2007, 37, 1329–1341. [Google Scholar] [CrossRef] [PubMed]
- Salcedo, S.P.; Holden, D.W. Bacterial interactions with the eukaryotic secretory pathway. Curr. Opin. Microbiol. 2005, 8, 92–98. [Google Scholar] [CrossRef] [PubMed]
- Coppens, I. Contribution of host lipids to Toxoplasma pathogenesis. Cell. Microbiol. 2006, 8, 1–9. [Google Scholar] [CrossRef] [PubMed]
- Coppens, I.; Sinai, A.P.; Joiner, K.A. Toxoplasma gondii Exploits Host Low-Density Lipoprotein Receptor-Mediated Endocytosis for Cholesterol Acquisition. J. Cell Biol. 2000, 149, 167–180. [Google Scholar] [CrossRef] [PubMed]
- Coppens, I.; Dunn, J.D.; Romano, J.D.; Pypaert, M.; Zhang, H.; Boothroyd, J.C.; Joiner, K.A. Toxoplasma gondii Sequesters Lysosomes from Mammalian Hosts in the Vacuolar Space. Cell 2006, 125, 261–274. [Google Scholar] [CrossRef] [PubMed]
- Joiner, K.A.; Fuhrman, S.A.; Miettinen, H.M.; Kasper, L.H.; Mellman, I. Toxoplasma gondii: Fusion Competence of Parasitophorous Vacuoles in Fc Receptor-Transfected Fibroblasts. Science 1990, 249, 641–646. [Google Scholar] [CrossRef] [PubMed]
- Mordue, D.G.; Håkansson, S.; Niesman, I.; Sibley, L.D. Toxoplasma gondii Resides in a Vacuole That Avoids Fusion with Host Cell Endocytic and Exocytic Vesicular Trafficking Pathways. Exp. Parasitol. 1999, 92, 87–99. [Google Scholar] [CrossRef]
- Mordue, D.G.; Sibley, L.D. Intracellular fate of vacuoles containing Toxoplasma gondii is determined at the time of formation and depends on the mechanism of entry. J. Immunol. 1997, 159, 4452–4459. [Google Scholar] [CrossRef]
- Mueller, A.-K.; Camargo, N.; Kaiser, K.; Andorfer, C.; Frevert, U.; Matuschewski, K.; Kappe, S.H.I. Plasmodium liver stage developmental arrest by depletion of a protein at the parasite–host interface. Proc. Natl. Acad. Sci. 2005, 102, 3022–3027. [Google Scholar] [CrossRef]
- Mueller, A.-K.; Labaied, M.; Kappe, S.H.I.; Matuschewski, K. Genetically modified Plasmodium parasites as a protective experimental malaria vaccine. Nature 2004, 433, 164–167. [Google Scholar] [CrossRef] [PubMed]
- Mikolajczak, S.A.; Jacobs-Lorena, V.; MacKellar, D.C.; Camargo, N.; Kappe, S.H. L-FABP is a critical host factor for successful malaria liver stage development. Int. J. Parasitol. 2007, 37, 483–489. [Google Scholar] [CrossRef] [PubMed]
- Piel, J.; Hui, D.; Fusetani, N.; Matsunaga, S. Targeting modular polyketide synthases with iteratively acting acyltransferases from metagenomes of uncultured bacterial consortia. Environ. Microbiol. 2004, 6, 921–927. [Google Scholar] [CrossRef] [PubMed]
- Sims, J.W.; Schmidt, E.W. Thioesterase-Like Role for Fungal PKS-NRPS Hybrid Reductive Domains. J. Am. Chem. Soc. 2008, 130, 11149–11155. [Google Scholar] [CrossRef] [PubMed]
- Yu, D.; Xu, F.; Zeng, J.; Zhan, J. Type III polyketide synthases in natural product biosynthesis. IUBMB Life 2012, 64, 285–295. [Google Scholar] [CrossRef] [PubMed]
- Rein, K.S.; Snyder, R.V. The biosynthesis of polyketide metabolites by dinoflagellates. Advances in applied microbiology 2006, 59, 93–125. [Google Scholar] [PubMed]
- Van Vranken, J.G.; Nowinski, S.M.; Clowers, K.J.; Jeong, M.Y.; Ouyang, Y.; Berg, J.A.; Rutter, J. ACP acylation is an acetyl-CoA-dependent modification required for electron transport chain assembly. Molecular cell 2018, 71, 567–580. [Google Scholar] [CrossRef] [PubMed]
- Kajikawa, M.; Yamato, K.T.; Kanamaru, H.; Sakuradani, E.; Shimizu, S.; Fukuzawa, H.; Sakai, Y.; Ohyama, K. MpFAE3, a β-Ketoacyl-CoA Synthase Gene in the LiverwortMarchantia polymorphaL., Is Preferentially Involved in Elongation of Palmitic Acid to Stearic Acid. Biosci. Biotechnol. Biochem. 2003, 67, 1667–1674. [Google Scholar] [CrossRef] [PubMed]
- Waller, R.F.; Ralph, S.A.; Reed, M.B.; Su, V.; Douglas, J.D.; Minnikin, D.E.; Cowman, A.F.; Besra, G.S.; McFadden, G.I. A Type II Pathway for Fatty Acid Biosynthesis Presents Drug Targets in Plasmodium falciparum. Antimicrob. Agents Chemother. 2003, 47, 297–301. [Google Scholar] [CrossRef] [PubMed]
- Burgess, S.C.; He, T.; Yan, Z.; Lindner, J.; Sherry, A.D.; Malloy, C.R.; Browning, J.D.; Magnuson, M.A. Cytosolic Phosphoenolpyruvate Carboxykinase Does Not Solely Control the Rate of Hepatic Gluconeogenesis in the Intact Mouse Liver. Cell Metab. 2007, 5, 313–320. [Google Scholar] [CrossRef]
- Tomova, C.; Humbel, B.M.; Geerts, W.J.C.; Entzeroth, R.; Holthuis, J.C.M.; Verkleij, A.J. Membrane Contact Sites between Apicoplast and ER in Toxoplasma gondii Revealed by Electron Tomography. Traffic 2009, 10, 1471–1480. [Google Scholar] [CrossRef] [PubMed]
- Ralph, S.A.; van Dooren, G.G.; Waller, R.F.; Crawford, M.J.; Fraunholz, M.J.; Foth, B.J.; Tonkin, C.J.; Roos, D.S.; McFadden, G.I. Metabolic maps and functions of the Plasmodium falciparum apicoplast. Nat. Rev. Microbiol. 2004, 2, 203–216. [Google Scholar] [CrossRef] [PubMed]
Figure 1.
Diagrammatic illustration of the metabolic pathways in Plasmodium falciparum cells that PfMQO is involved with. Abbreviations, Q: ubiquinone; QH2: ubiquinol; FH: fumarate hydratase; AAT: aspartate aminotransferase; MDH: malate dehydrogenase.
Figure 1.
Diagrammatic illustration of the metabolic pathways in Plasmodium falciparum cells that PfMQO is involved with. Abbreviations, Q: ubiquinone; QH2: ubiquinol; FH: fumarate hydratase; AAT: aspartate aminotransferase; MDH: malate dehydrogenase.
Figure 2.
Schematic representation of an acidocalcisome. Cl-, Na+/H+, Ca2+/H+, Aquaporins (water channels), amino acids transporters are established for the exchange of these crucial ions as well as the amino acids into the matrix of acidocalcisome matrix. Major channels however are the vacuolar ATPase (V-H+-ATPase), Ca2+ ATPase and vacuolar pyrophosphatase (V-H+-PPase). Also present are the transporters for basic amino acids, cations, phosphates (Pi), pyrophosphates (PPi), polyphosphate (poly P) etc., thus enriching the matrix of the acidocalcisome with Pi, PPi, polyp and also with enzymes that are critical in the metabolism of poly P, such as poly P kinase (PPK), poly P pyrophosphatase (PPase) and poly P exopolyphosphatase (PPX). PMCA, plasma membrane-type Ca2+-ATPase. VDAC, voltage-dependent anion-selective channel. IP3R, inositol 1,4,5-trisphosphate receptor. Different acidocalcisomes contains different assortment of these enzymes (75). This diagram also shows the molecules involve in Ca2+ detection at the intermembrane space, Ca2+ influx (MCU), and Ca2+ efflux across the inner mitochondrial membrane. A more elaborate work by Docampo, in his work “the origin and evolution of the acidocalcisome and its interactions with other organelles” provides a greater inside to acidocalcisome (75). A genome-scale analysis of the isometamidium resistance of T. Brucei revealed that the interaction between the acalcisomes and mitochondria could be a potential mechanism of drug resistance. The screening revealed that numerous genes are involved in the acalcisomes’ production as well as the four AP-3 subunits, which were implicated in the resistance (76). The screening revealed that the reduction of the expression of two of V-ATPase’s subunits by RNAi led to an increase in its resistance. This resistance was validated by the screening results. In addition, the RNAi downregulation of other subunits caused resistance to various drugs, such as berenil and ethidium bromide (76,77). After analyzing the RNAi of V-ATPase, it was concluded that the accumulation of isometamidium did not contribute to the resistance, this was carried out using fluorescence shifts detection. The exhaustion of the AP-3, V-ATPase, and EMC components by the use of tetracycline also increased their resistance to acriflavin. However, the removal of the antibiotic caused the cells to die, which indicated that the depletion of these subunits could allow T. Brucei to tolerate diskinethoplasty. In addition, depletion of V-ATPase likewise amplified its resistance to the growth inhibition of oligomycin (an inhibitor of the mitochondrial ATP synthase). The authors hypothesized that the activity of V-ATPase maintains the ATP synthesis coupling in the cell (76).
Figure 2.
Schematic representation of an acidocalcisome. Cl-, Na+/H+, Ca2+/H+, Aquaporins (water channels), amino acids transporters are established for the exchange of these crucial ions as well as the amino acids into the matrix of acidocalcisome matrix. Major channels however are the vacuolar ATPase (V-H+-ATPase), Ca2+ ATPase and vacuolar pyrophosphatase (V-H+-PPase). Also present are the transporters for basic amino acids, cations, phosphates (Pi), pyrophosphates (PPi), polyphosphate (poly P) etc., thus enriching the matrix of the acidocalcisome with Pi, PPi, polyp and also with enzymes that are critical in the metabolism of poly P, such as poly P kinase (PPK), poly P pyrophosphatase (PPase) and poly P exopolyphosphatase (PPX). PMCA, plasma membrane-type Ca2+-ATPase. VDAC, voltage-dependent anion-selective channel. IP3R, inositol 1,4,5-trisphosphate receptor. Different acidocalcisomes contains different assortment of these enzymes (75). This diagram also shows the molecules involve in Ca2+ detection at the intermembrane space, Ca2+ influx (MCU), and Ca2+ efflux across the inner mitochondrial membrane. A more elaborate work by Docampo, in his work “the origin and evolution of the acidocalcisome and its interactions with other organelles” provides a greater inside to acidocalcisome (75). A genome-scale analysis of the isometamidium resistance of T. Brucei revealed that the interaction between the acalcisomes and mitochondria could be a potential mechanism of drug resistance. The screening revealed that numerous genes are involved in the acalcisomes’ production as well as the four AP-3 subunits, which were implicated in the resistance (76). The screening revealed that the reduction of the expression of two of V-ATPase’s subunits by RNAi led to an increase in its resistance. This resistance was validated by the screening results. In addition, the RNAi downregulation of other subunits caused resistance to various drugs, such as berenil and ethidium bromide (76,77). After analyzing the RNAi of V-ATPase, it was concluded that the accumulation of isometamidium did not contribute to the resistance, this was carried out using fluorescence shifts detection. The exhaustion of the AP-3, V-ATPase, and EMC components by the use of tetracycline also increased their resistance to acriflavin. However, the removal of the antibiotic caused the cells to die, which indicated that the depletion of these subunits could allow T. Brucei to tolerate diskinethoplasty. In addition, depletion of V-ATPase likewise amplified its resistance to the growth inhibition of oligomycin (an inhibitor of the mitochondrial ATP synthase). The authors hypothesized that the activity of V-ATPase maintains the ATP synthesis coupling in the cell (76).
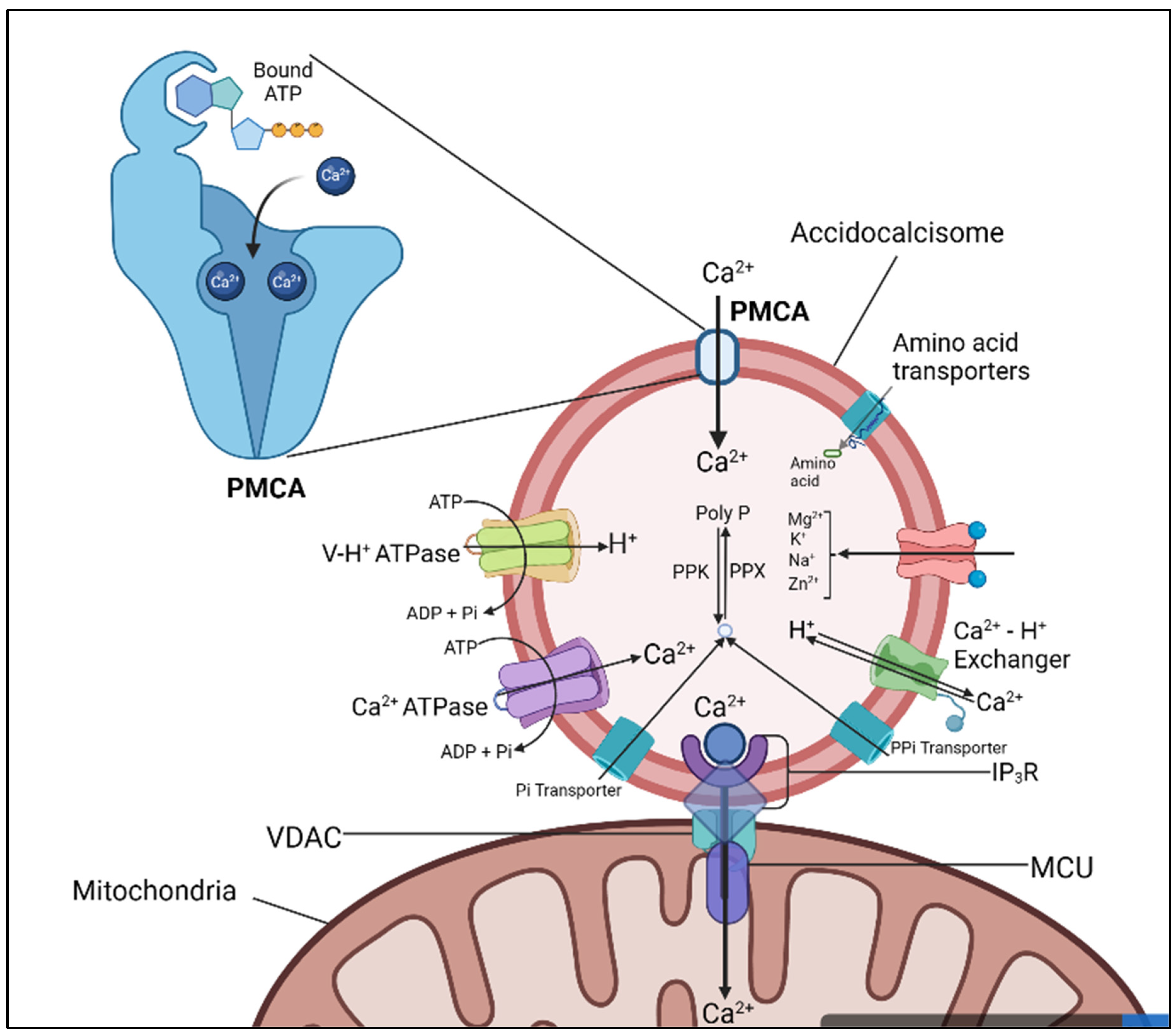
Figure 3.
Diagram showing the mechanisms of Apicomplexans uptake of fatty acid. (a) Host cell’s lysosomal vesicles (source of sterols and fatty acids) trapped by Toxoplasma in the parasitophorous vacuole (PV), the process is mediated by the parasite’s protein GRA7. (b) and (c) The schizont of Plasmodium in the liver schizont produces UIS3 that targets PV membrane and binds to host cell fatty acid binding protein (FABP) thus aiding in fatty acid uptake via direct diffusion or via PV pores or other transporters. (d)The parasite might secrete factors into the PV to encourage the recruitment of mitochondria of the host cell in order to salvage fatty acids and lipids. (e) The parasite might secrete proteins into the PV that enhances the recruitment of endoplasmic reticulum (ER) to the PV’s membrane, thus enhancing fatty acids uptake. (f) Another Apicomplexan, especially the Cryptosporidium is known to secrete acyl-CoA binding protein (ACBP) into the PV, which is critical in binding acyl-CoA.
Figure 3.
Diagram showing the mechanisms of Apicomplexans uptake of fatty acid. (a) Host cell’s lysosomal vesicles (source of sterols and fatty acids) trapped by Toxoplasma in the parasitophorous vacuole (PV), the process is mediated by the parasite’s protein GRA7. (b) and (c) The schizont of Plasmodium in the liver schizont produces UIS3 that targets PV membrane and binds to host cell fatty acid binding protein (FABP) thus aiding in fatty acid uptake via direct diffusion or via PV pores or other transporters. (d)The parasite might secrete factors into the PV to encourage the recruitment of mitochondria of the host cell in order to salvage fatty acids and lipids. (e) The parasite might secrete proteins into the PV that enhances the recruitment of endoplasmic reticulum (ER) to the PV’s membrane, thus enhancing fatty acids uptake. (f) Another Apicomplexan, especially the Cryptosporidium is known to secrete acyl-CoA binding protein (ACBP) into the PV, which is critical in binding acyl-CoA.
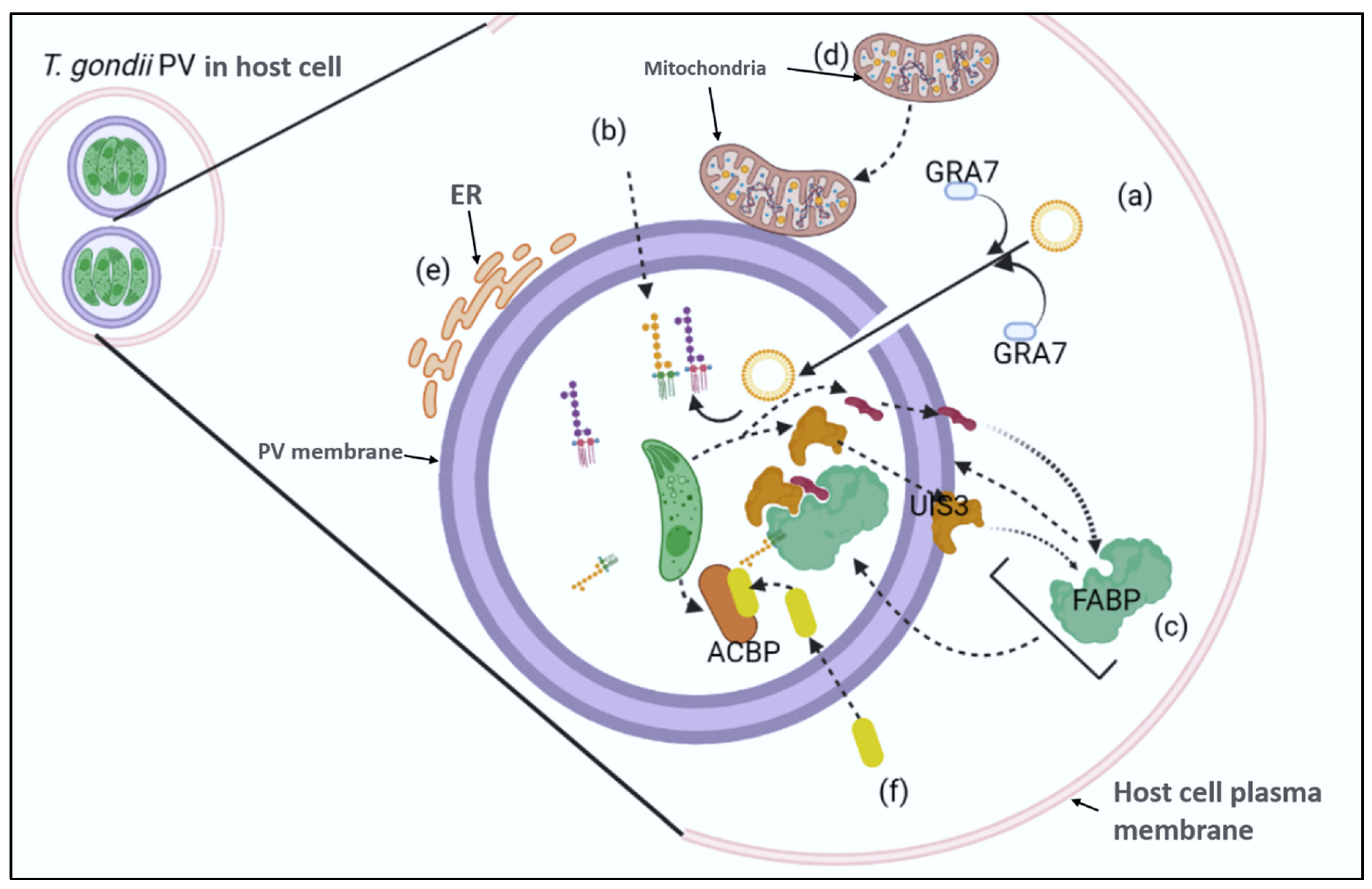
|
Disclaimer/Publisher’s Note: The statements, opinions and data contained in all publications are solely those of the individual author(s) and contributor(s) and not of MDPI and/or the editor(s). MDPI and/or the editor(s) disclaim responsibility for any injury to people or property resulting from any ideas, methods, instructions or products referred to in the content. |
© 2023 by the authors. Licensee MDPI, Basel, Switzerland. This article is an open access article distributed under the terms and conditions of the Creative Commons Attribution (CC BY) license (http://creativecommons.org/licenses/by/4.0/).