1. Introduction
Parkinson’s disease (PD) is the second most common neurodegenerative disorder, afflicting 1-2% of adults over age 65 worldwide [
1]. PD is pathologically characterized by the progressive loss of dopaminergic neurons in the
substantia nigra pars compacta (SNpc) accompanied by the misfolding of α-syn into beta sheets, forming Lewy bodies in the midbrain. Mitochondrial dysfunction is an important factor contributing to PD, occurring when there is an imbalance among reactive oxygen species (ROS) and antioxidants, resulting in cellular stress. Inhibition of complex I in the mitochondrial electron transport chain induces enhanced ROS generation [
2], ultimately inhibiting activity of the proteasome, lysosomes, and mitochondria [
3]. Further, enhanced cellular ROS promotes the misfolding of α-syn and perturbs protein clearance mechanisms, exacerbating disease [
4]. Such pathologies have been found in the SNpc of PD patients [
7,
8].
Unfortunately, many hallmark symptoms of PD such as bradykinesia, tremor, and abnormal gait remain undetectable until a substantial amount of dopaminergic cell death has occurred [
7]. Despite robust efforts to develop therapeutics for PD, treatment remains focused on the alleviation of symptoms rather than ceasing disease progression. Moreover, the exact pathology underlying the foundation of this disease remains unclear. To date, twenty genes have been deemed causative of PD, while genome wide association studies have identified 90 variants associated with PD risk [
8]. However, the majority of PD cases are idiopathic with disease spurring from interactions between genetic networks and environmental factors [
9].
Adenosine to inosine (A-to-I) editing is the mechanism in which adenosine is converted to inosine in regions of double-stranded RNA; this base change is catalyzed by a family of enzymes known as adenosine deaminases that act on RNA (ADARs) [
10]. The chemical properties of inosine are similar to those of guanosine, so it is treated as guanosine by translational machinery [
11]. RNA editing by ADARs profoundly increases transcriptomic and proteomic diversity. ADARs are imperative for the healthy development and function of the nervous system [
12], and ADAR protein dysfunction has been implicated in a variety of neurological diseases including amyotrophic lateral sclerosis, epilepsy, developmental epileptic encephalopathy, and Aicardi-Goutières syndrome, among others [
13]. Furthermore, knockouts of ADAR genes in model organisms have exhibited profound neurological dysfunction. For example, ADAR2 knockout mice are prone to seizures and have a shortened lifespan [
14], and
Adar mutant
Drosophila display synaptic and neurotransmission defects [
15]. Notably, a recent study in post-mortem brain tissue revealed differential editing in mRNA of PD patients that succumbed to disease [
16]. Thus, it is plausible that ADARs have a salient role in PD.
The nematode roundworm,
C. elegans, is an exceptional model organism to study progressive neurodegenerative diseases such as PD due to its hermaphroditic reproduction, large brood size, short generation time (~3 days) and ease of maintenance [
17,
18]. Additionally, the entire connectome of
C. elegans has been delineated [
19]. Thus,
C. elegans provides researchers with the ability to rapidly analyze isogenic populations and precisely quantify neurodegeneration in individuals disadvantaged by α-syn, the central protein associated with dopaminergic neurodegeneration in PD. In
C. elegans, two ADAR genes with human orthologs have been identified,
adr-1 and
adr-2. Analyses of these genes indicated that both have distinct roles in RNA editing and are essential for normal nervous system function in
C. elegans [
20]. However, ADR-2 is the only active adenosine deaminase, as knockout of
adr-2 abolishes all A-to-I RNA editing [
21]. In contrast
, ADR-1 acts as a regulator of ADR-2 by facilitating the binding of ADR-2 to its targets [
21,
22]. Previous work on ADARs in
C. elegans has resulted in the identification of genes that are both regulated and edited by ADR-2, including
xdh-1 and
wht-2, respectively [
23].
Xanthine dehydrogenase (XDH) is an enzyme that is responsible for the catabolism of hypoxanthine to xanthine and xanthine to uric acid, the final two steps in the purine catabolic pathway [
24,
25]. Located intracellularly in peroxisomes and the cytosol, XDH has two subunits, each consisting of four redox center domains: a molybdopterin center, two iron sulfur centers, a flavin adenine dinucleotide (FAD) center, and two iron-sulfur subunits [
26]. NADH and uric acid are produced as byproducts of XDH activity. Additionally, XDH can be converted to xanthine oxidase (XO) both irreversibly and reversibly via proteolysis or the oxidation of two cysteine residues, respectively. This interconversion occurs in the presence of urea or when concentrations of guanidine hydrochloride are low. Oxygen is required for XO activity in lieu of NAD, yielding superoxide anion, hydrogen peroxide, and uric acid [
26]. This difference in cofactors is attributed to the capacity for XDH to rapidly react with NAD and slowly with oxygen, while XO does the opposite [
27]. This stems from the conformational change that results upon XDH to XO conversion—in simplest terms, the XDH has a higher binding affinity than XO [
26]. As a result, XO is a major generator of ROS (superoxide anion and hydrogen peroxide), which significantly contributes to oxidative stress.
ATP binding cassette subfamily G member 2 (ABCG2), the human ortholog of worm WHT-2, is an efflux transporter containing a hydrophobic transmembrane domain and a nucleotide binding domain [
28]. It can be found in various tissues such as the intestine, kidney and brain. Specifically, it is expressed on the apical side of endothelial cells in the blood brain barrier (BBB). It has also been shown to be located in mitochondrial cristae [
29]. ABCG2 is a transporter of many endogenous substrates such as urate, steroids, and heme, as well as xenobiotic compounds. Importantly, it has been shown to be upregulated in response to oxidative stress as it is able to efflux substances that generate ROS [
30].
Here, we show experimental evidence that reduction of functional XDH in dopamine neurons is protective against neurodegeneration attributed to oxidative stress induced by α-syn. Further, we propose a network in which ADR-2 edits WHT-2/ABCG2, altering its structure and, thus, its ability to export uric acid from the cell. In turn, XDH is downregulated to maintain homeostasis and prevent potential deleterious effects of excess uric acid.
4. Discussion
Despite the lack of complete understanding of the causative factors of PD, it is accepted that sporadic PD cases arise because of genetic and environmental factors. Indeed, rigorous work in both the clinical and laboratory settings have convincingly connected PD with processes such as oxidative stress and mitochondrial dysfunction. Moreover, numerous studies in various cellular and organismal models have implicated ADARs in neurological diseases, but few have directly correlated RNA editing with disease. Our present study builds upon this body of evidence by connecting ADARs to disease pathologies in multiple C. elegans models of PD, and by predicting the impact of editing at the protein level on α-syn protein misfolding and α-syn-induced neurodegeneration.
We have demonstrated that several genes regulated by ADARs are involved in α-syn misfolding and dopaminergic neuronal cell death, two pathological hallmarks of PD. We have shown that knockdown of these genes altered the misfolding of α-syn, and such modifiers of protein misfolding were most highly associated with FAD and iron ion binding. When knocked down in the dopamine neurons of worms co-expressing α-syn and GFP under the control of the P
dat-1 promoter, four presumptive modifiers of protein misfolding altered neurodegeneration:
xdh-1, acdh-1, pho-1,
F52E1.2 and
papl-1. All these genes were protective when knocked down except
papl-1, which enhanced dopaminergic neuronal cell death. Consistent with recent reports suggesting that monomeric and oligomeric α-syn is more cytotoxic than the aggregated form [
57,
58], the genes that were neuroprotective enhanced protein misfolding when knocked down. Further, we have shown that knockout of
xdh-1, which conferred the strongest observed effect upon knockdown, was indeed protective in our neuronal PD model. Finally, we illustrate that WHT-2, which interacts with XDH-1 and is edited by ADR-2, appears to act in the same pathway as XDH-1 to protect dopaminergic neurons from α-syn-induced neurotoxicity. Concurrent knockdown of
xdh-1 and
wht-2 by RNAi indicates that the neuroprotection associated with loss or absence of
xdh-1 is not contingent on
adr-2 expression. Thus, we propose a model in which the absence of editing leads to ineffective WHT-2 export of uric acid, which is produced by XDH-1, in turn reducing the toxicity of α-syn and protecting dopaminergic neurons from degeneration (
Figure 6).
XDH, the human ortholog of XDH-1, is responsible for the breakdown of hypoxanthine to xanthine and xanthine to uric acid, the final two steps of purine catabolism. Uric acid has been associated with neuroprotection, as it serves as a scavenger of free radicals, in turn protecting against ROS. Notably, higher serum urate has been associated with lower risk of PD and slower progression of PD [
59]. Additionally, it has been demonstrated that uric acid inhibits ROS accumulation and improves mitochondrial function in rat hippocampal neurons [
60]. Further, uric acid is a powerful iron chelator, and dysregulation with iron metabolism has been correlated with oxidative stress in PD [
61]. Drugs that promote uric acid and iron accumulation have shown moderate success in clinical trials, but both strategies for treating PD have resulted in iatrogenic effects. For instance, uric acid accretion has resulted in kidney stones containing uric acid crystals [
25,
62] and iron chelation has propagated anemia [
63]. Given that both iron and uric acid are associated with XDH activity, it is plausible that this enzyme is a promising therapeutic target for PD if its activity is regulated specifically in the SNpc.
ABCG2, the human ortholog of C. elegans WHT-2, exports uric acid, among many other endogenous and xenobiotic substrates. Our study illustrates that RNAi of wht-2 alone and wht-2 and xdh-1 simultaneously in dopamine neurons of worm expressing α-syn and GFP leads to comparable levels of neuroprotection. Thus, it is possible that XDH-1 activity is reduced to limit uric acid production when WHT-2 is dysfunctional, to avoid the deleterious effects of hyperuricemia. Both ABCG2 and XDH inhibition have been associated with hyperuricemia and gout, providing further evidence of our hypothesis that these proteins act in the same pathway. Moreover, simultaneous RNAi of both genes did not have an additive phenotypic effect in either of our PD models—additional affirmation of this conjecture.
Reports have indicated that the 3’ UTRs of many genes are edited by ADARs, as these regions contain Alu elements [
64]. ABCG2 is known to be regulated by various miRNAs, including at the 3’ UTR. One study in the S1 colon cancer cell line revealed that a miRNA binds to the 3’ UTR of ABCG2, thereby decreasing expression [
65]. miRNAs are known to repress translation, through various modes of regulation including preventing translational initiation, which does not necessarily impact transcription of the target gene [
66]. It is possible that editing by ADARs inhibits the binding of this miRNA to the 3’ UTR of ABCG2, leading to increased ABCG2 translation. Such events could lead to an increase of XDH activity, as more uric acid produced by XDH could be excreted out of the cell by ABCG2. However, in the absence of ADAR activity, the putative miRNA can bind to the gene encoding ABCG2, leading to reduced translation of ABCG2 and the dampening of uric acid transport out of the cell. This would potentially lead to a decrease in XDH expression to maintain cellular uric acid homeostasis (
Figure 6). This prospective mechanism would explain why
xdh-1 is downregulated upon loss of
adr-2 in
C. elegans, while
wht-2 mRNA levels are not altered [
25]. Of course, this hypothesis must be validated experimentally.
It is interesting that knockdown of
xdh-1 and
wht-2 lead to enhanced neuroprotection and increased protein misfolding. It is possible that the intracellular elevation in uric acid is protective against dopaminergic neuronal cell death by increasing the aggregation of α-syn, the less cytotoxic form of this protein. However, whether increased misfolding is associated with enhanced or diminished disease progression remains controversial. It has been shown that soluble α-syn monomers and oligomers, which precede fibril formation, are more cytotoxic than larger inclusions due to their propensity to disrupt cell membrane permeability and promote neuroinflammation [
57]. Further, analysis of post-mortem brain tissue has indicated that more monomers and oligomers are found in PD patients in comparison to age-matched controls [
67]. Contrastingly, other researchers have associated increased protein misfolding with more severe disease [
68,
69]. While our results suggest that enhanced misfolding of α-syn is commensurate to increased dopaminergic neuronal health, these data are due to gene expression in the body wall muscle. RNAi of
xdh-1 was cell autonomous, and it is possible that RNAi exclusively in the body wall may yield different results. The generation of a model that expresses α-syn and GFP in the body wall muscles and is RNAi sensitive solely in the body wall muscles, in addition to more studies on the role of α-syn misfolding in PD, have the potential to resolve this issue.
Notably, loss of ADR-2 activity did not impact α-syn-induced neurodegeneration, especially due to supporting evidence that aberrant ADAR activity has detrimental neurological effects across species, including
C. elegans. For example, it has been shown that
C. elegans that lack editing by ADARs exhibit reduced chemotaxis [
23]. However, chemotaxis in
C. elegans is regulated by ASE sensory neurons, not dopaminergic neurons [
70]. Since dopamine neurons were the only neuronal cell type evaluated in our study, it is possible that aberrant ADR-2 activity does indeed impact the health of other neuronal cell types. Further, it is surprising that loss of ADR-2 did not impact aggregation of α-syn. This may be due to a lack of expression of
adr-2 in the body wall—high levels of ADR-2 activity have been reported in the
C. elegans nervous system specifically [
22,
25], but expression patterns in other tissues have not been extensively studied. It is also possible that the absence of observable phenotypes attributable to
adr-2 deletion in neurons and body wall muscles expressing α-syn may be an additive effect of changes in expression of all targets of ADARs—simply put, some genes may lead to enhanced neurodegeneration or misfolding, or vice versa, cumulatively leading to no net change.
Here, we describe a relationship between ADR-2, WHT-2, and XDH-1 that impacts PD pathologies. Further, we identify precisely how RNA editing by ADARs affects the folding of the WHT-2 protein, presumably changing
xdh-1 expression. While additional work is needed to further elucidate this mechanism, the targeting of ABCG2, the human ortholog of WHT-2, by ADARs may serve as a promising therapeutic strategy for the treatment of PD. Because ADARs target many genes in different tissues, the targeted altering of editing or edited substrate proteins, opposed to a complete abolishment of editing, is presumably a better approach. Nonetheless, RNA editing represents an exciting area of enquiry with substantial implications for biomedical research. The foundational studies on ADAR function in
C. elegans have established this system as an exceptional model for investigation of these transformative enzymes as putative modifying factors in neurological diseases such as PD [
20,
71]. Moreover, the cost-effective application of
C. elegans towards translational goals is expanding as an outcome of the increasingly proven preclinical utility of worm disease models [
72,
73,
74].
Author Contributions
For research articles with several authors, a short paragraph specifying their individual contributions must be provided. The following statements should be used “Conceptualization, L.A.S., L.A.B., K.A.C. and G.A.C.; methodology, L.A.S., L.A.B., K.A.C. and G.A.C..; software, L.A.S.; validation, L.A.S., K.A.C., and G.A.C..; formal analysis, L.A.S., K.A.C. and G.A.C.; investigation, L.A.S., L.E.M., K.P., and L.M.S. ; resources, K.A.C. and G.A.C..; data curation; writing—original draft preparation, L.A.S..; writing—review and editing, L.A.S., K.A.C., and G.A.C.; visualization, L.A.S. and K.A.C..; supervision, K.A.C. and G.A.C..; project administration, L.A.S., K.A.C., and G.A.C..; funding acquisition, L.A.S., L.E.M., K.A.C., G.A.C. All authors have read and agreed to the published version of the manuscript.
Figure 1.
RNAi of genes differentially regulated in adr-2 mutants alter protein misfolding. (A) Isogenic worm strain expressing α-syn::GFP in the body wall muscle cells of C. elegans were fed E. coli expressing candidate gene RNAi constructs and scored for aggregate size and number, which were combined to generate an overall aggregate score. Synchronized worms were analyzed two days post-hatch, and a percent change for each gene was calculated in comparison to empty vector control. The top 14 genes that resulted in the greatest cumulative percent change in aggregation are shown in red. (B) RNAi of xdh-1 (bottom) in worms expressing α-syn::GFP in the body wall muscles under control of the Punc-54 promoter showed increased aggregate size and number in comparison to empty vector control (top). Examples of scoring criteria: A = small aggregate, B = medium aggregate, C = large aggregate, D = nuclei of body wall muscle cell.
Figure 1.
RNAi of genes differentially regulated in adr-2 mutants alter protein misfolding. (A) Isogenic worm strain expressing α-syn::GFP in the body wall muscle cells of C. elegans were fed E. coli expressing candidate gene RNAi constructs and scored for aggregate size and number, which were combined to generate an overall aggregate score. Synchronized worms were analyzed two days post-hatch, and a percent change for each gene was calculated in comparison to empty vector control. The top 14 genes that resulted in the greatest cumulative percent change in aggregation are shown in red. (B) RNAi of xdh-1 (bottom) in worms expressing α-syn::GFP in the body wall muscles under control of the Punc-54 promoter showed increased aggregate size and number in comparison to empty vector control (top). Examples of scoring criteria: A = small aggregate, B = medium aggregate, C = large aggregate, D = nuclei of body wall muscle cell.
Figure 2.
ADR-2-regulated candidate modifiers of α-syn misfolding are most highly associated with FAD and iron ion binding and impact dopamine neuronal health.
(A) Graphical representation of biological processes (
www.wormbase.org), molecular functions and cellular components associated with genes differentially regulated in adr-2 mutants and alter α-syn misfolding.
(B) GO terms enriched in candidate genes
(C) Candidate genes were knocked down in dopaminergic neurons of C. elegans expressing α-syn and GFP under control of the P
dat-1 promoter. Subsequent scoring of dopaminergic neuronal health and worm population analysis indicate that RNAi of xdh-1, acdh-1, pho-1 and F52E1.2 were protective against α-syn induced dopaminergic neurodegeneration, while papl-1 knockdown enhanced dopaminergic neuronal death. Worms were analyzed as 7-day old adults. Chi-Square analysis was performed where each candidate gene knockdown value was compared to EV and are represented by exact p values (three independent experiments, n = 30 per independent groups; N=3; n=30).
Figure 2.
ADR-2-regulated candidate modifiers of α-syn misfolding are most highly associated with FAD and iron ion binding and impact dopamine neuronal health.
(A) Graphical representation of biological processes (
www.wormbase.org), molecular functions and cellular components associated with genes differentially regulated in adr-2 mutants and alter α-syn misfolding.
(B) GO terms enriched in candidate genes
(C) Candidate genes were knocked down in dopaminergic neurons of C. elegans expressing α-syn and GFP under control of the P
dat-1 promoter. Subsequent scoring of dopaminergic neuronal health and worm population analysis indicate that RNAi of xdh-1, acdh-1, pho-1 and F52E1.2 were protective against α-syn induced dopaminergic neurodegeneration, while papl-1 knockdown enhanced dopaminergic neuronal death. Worms were analyzed as 7-day old adults. Chi-Square analysis was performed where each candidate gene knockdown value was compared to EV and are represented by exact p values (three independent experiments, n = 30 per independent groups; N=3; n=30).
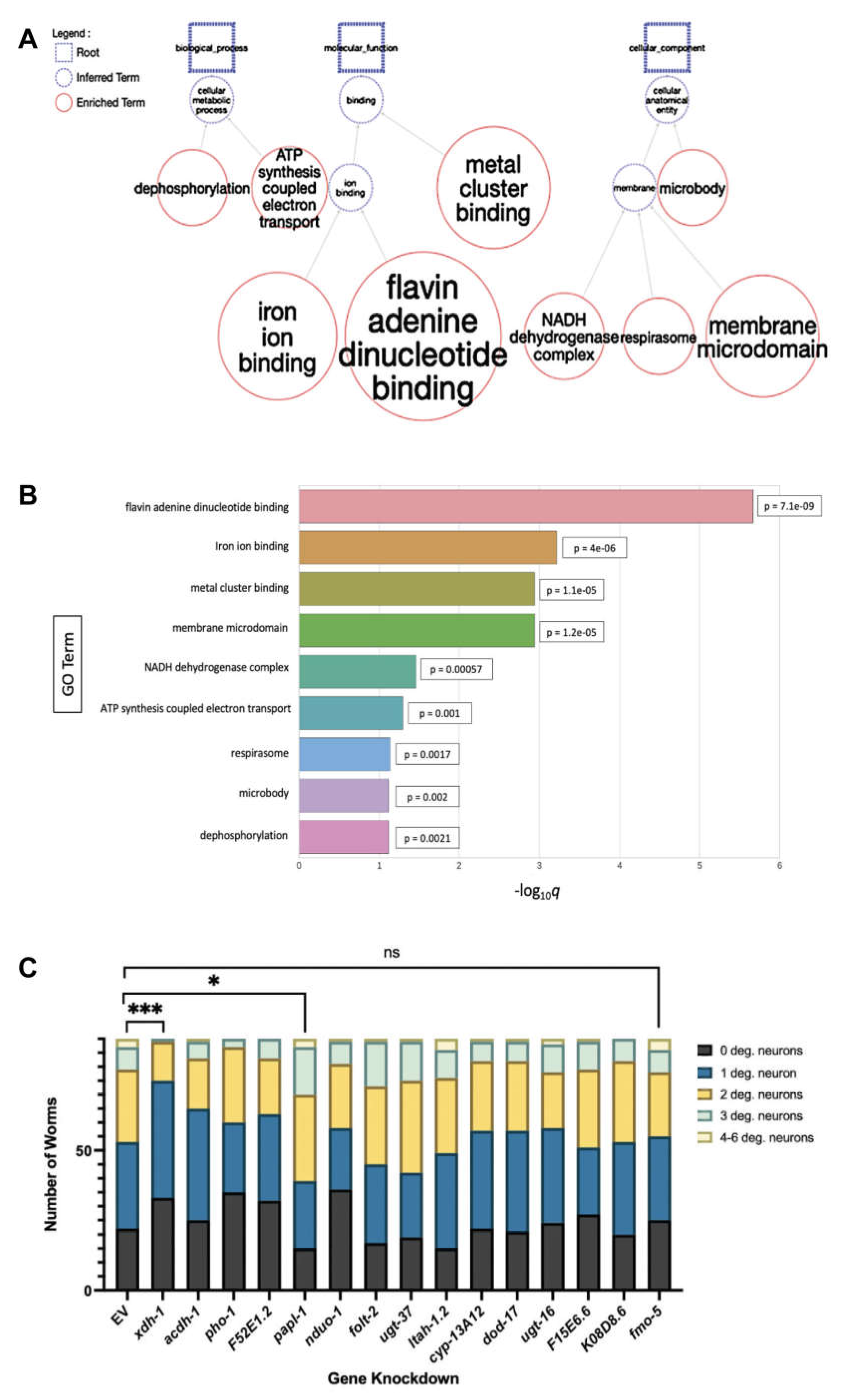
Figure 3.
XDH-1 modulates neuroprotection by impacting ROS production. (A) C. elegans expressing α-syn + GFP in dopaminergic neurons, wildtype, and mutant for xdh-1. Arrows represent wildtype dopamine neurons with a cell body and intact axonal process, and arrowheads represent degenerated dopamine neurons. The xdh-1 mutation confers protection against α-syn induced neurodegeneration. (B-C) Animals with wildtype or mutant xdh-1 were crossed to isogenic worms expressing α-syn + GFP in dopamine neurons. The four CEP and two ADE dopaminergic neurons were analyzed for the presence or absence of intact cell bodies and axonal processes in synchronized worms seven days post-hatch (three independent experiments, n = 30 per independent groups). Worms with the xdh-1(ok3134) mutation exhibit neuroprotection against α-syn. (B) The number of degenerated neurons in each individual worm of the total population. Chi-Square analysis was performed where α-syn + xdh-1(ok3134) was compared to α-syn alone and values are represented by exact p values (three independent experiments, n = 30 per independent groups; N=3; n=30). (C) The percent of worms with 6 normal dopaminergic neurons. Student’s t-test where α-syn + xdh-1(ok3134) was compared to α-syn alone and values are represented by exact p values (three independent experiments, n = 30 per independent groups; N=3; n=30). (D-E) Worms overexpressing xdh-1 under control of the Pdat-1 promoter were crossed to worms expressing α-syn + GFP in dopaminergic neurons. Subsequently, animals were synchronized and scored for neurodegeneration. Worms with increased xdh-1 expression in dopaminergic neurons showed decreased neuroprotection four days post-hatch. (D) The number of degenerated neurons in each individual worm of the total population. Chi square analysis was performed where α-syn + Pdat-1::xdh-1 was compared to α-syn alone and values are represented by exact p values (three independent experiments, n = 30 per independent groups; N=3; n=30). (E) The percent of worms with 6 normal dopaminergic neurons. Student’s t-test where α-syn + Pdat-1::xdh-1 was compared to α-syn alone and values are represented by exact p values (three independent experiments, n = 30 per independent groups; N=3; n=30). (F) In the purine catabolic pathway, xanthine dehydrogenase (XDH) and xanthine oxidase (XO) catalyze hypoxanthine to xanthine and xanthine to uric acid. XDH and XO are interconvertible and are encoded by a single gene. For XDH activity, NAD is required as a cofactor, and NADH and uric acid are produced. In contrast, molecular oxygen is needed for XO to function, and uric acid and superoxide anions result. Reactive oxygen species (ROS) contribute to mitochondrial dysfunction, oxidative stress, and ultimately cell death. Created with Biorender.com. (G) Wildtype N2 worms, xdh-1(ok3134) worms, and worms expressing α-syn in dopaminergic neurons with and without the xdh-1(ok3134) mutant, were treated with a specialized fluorescein that moves into the cell and promotes the removal of acetate groups by cellular esterase. The product of this can be oxidized by ROS to form a fluorescent molecule to allow for quantification of ROS. Plate spectroscopy readouts were recorded at an excitation of 485nm and an emission of 525 nm every 15 minutes for a total of 2.5 hours. Normalization of DCF-DA signal to cell population was conducted prior to the start of the assay. Three biological replicates and two technical replicates were completed for all worm strains. After 2.5 hours, worms expressing α-syn and GFP in dopaminergic neurons that are mutant for xdh-1 produce lower ROS than worms without the mutation. Since ROS contribute to oxidative stress, mitochondrial dysfunction, and ultimately neuronal death, this decrease in ROS in the xdh-1(ok3134) mutant could contribute to neuroprotection. xdh-1 worms lacking α-syn exhibited enhanced ROS production at 2.5 hours, indicating that changes in ROS production due to XDH/XO activity is α-syn specific. Significance was obtained using a One-way ANOVA with a Tukey’s post hoc test and are represented by exact p values (N= 3; n=30).
Figure 3.
XDH-1 modulates neuroprotection by impacting ROS production. (A) C. elegans expressing α-syn + GFP in dopaminergic neurons, wildtype, and mutant for xdh-1. Arrows represent wildtype dopamine neurons with a cell body and intact axonal process, and arrowheads represent degenerated dopamine neurons. The xdh-1 mutation confers protection against α-syn induced neurodegeneration. (B-C) Animals with wildtype or mutant xdh-1 were crossed to isogenic worms expressing α-syn + GFP in dopamine neurons. The four CEP and two ADE dopaminergic neurons were analyzed for the presence or absence of intact cell bodies and axonal processes in synchronized worms seven days post-hatch (three independent experiments, n = 30 per independent groups). Worms with the xdh-1(ok3134) mutation exhibit neuroprotection against α-syn. (B) The number of degenerated neurons in each individual worm of the total population. Chi-Square analysis was performed where α-syn + xdh-1(ok3134) was compared to α-syn alone and values are represented by exact p values (three independent experiments, n = 30 per independent groups; N=3; n=30). (C) The percent of worms with 6 normal dopaminergic neurons. Student’s t-test where α-syn + xdh-1(ok3134) was compared to α-syn alone and values are represented by exact p values (three independent experiments, n = 30 per independent groups; N=3; n=30). (D-E) Worms overexpressing xdh-1 under control of the Pdat-1 promoter were crossed to worms expressing α-syn + GFP in dopaminergic neurons. Subsequently, animals were synchronized and scored for neurodegeneration. Worms with increased xdh-1 expression in dopaminergic neurons showed decreased neuroprotection four days post-hatch. (D) The number of degenerated neurons in each individual worm of the total population. Chi square analysis was performed where α-syn + Pdat-1::xdh-1 was compared to α-syn alone and values are represented by exact p values (three independent experiments, n = 30 per independent groups; N=3; n=30). (E) The percent of worms with 6 normal dopaminergic neurons. Student’s t-test where α-syn + Pdat-1::xdh-1 was compared to α-syn alone and values are represented by exact p values (three independent experiments, n = 30 per independent groups; N=3; n=30). (F) In the purine catabolic pathway, xanthine dehydrogenase (XDH) and xanthine oxidase (XO) catalyze hypoxanthine to xanthine and xanthine to uric acid. XDH and XO are interconvertible and are encoded by a single gene. For XDH activity, NAD is required as a cofactor, and NADH and uric acid are produced. In contrast, molecular oxygen is needed for XO to function, and uric acid and superoxide anions result. Reactive oxygen species (ROS) contribute to mitochondrial dysfunction, oxidative stress, and ultimately cell death. Created with Biorender.com. (G) Wildtype N2 worms, xdh-1(ok3134) worms, and worms expressing α-syn in dopaminergic neurons with and without the xdh-1(ok3134) mutant, were treated with a specialized fluorescein that moves into the cell and promotes the removal of acetate groups by cellular esterase. The product of this can be oxidized by ROS to form a fluorescent molecule to allow for quantification of ROS. Plate spectroscopy readouts were recorded at an excitation of 485nm and an emission of 525 nm every 15 minutes for a total of 2.5 hours. Normalization of DCF-DA signal to cell population was conducted prior to the start of the assay. Three biological replicates and two technical replicates were completed for all worm strains. After 2.5 hours, worms expressing α-syn and GFP in dopaminergic neurons that are mutant for xdh-1 produce lower ROS than worms without the mutation. Since ROS contribute to oxidative stress, mitochondrial dysfunction, and ultimately neuronal death, this decrease in ROS in the xdh-1(ok3134) mutant could contribute to neuroprotection. xdh-1 worms lacking α-syn exhibited enhanced ROS production at 2.5 hours, indicating that changes in ROS production due to XDH/XO activity is α-syn specific. Significance was obtained using a One-way ANOVA with a Tukey’s post hoc test and are represented by exact p values (N= 3; n=30).
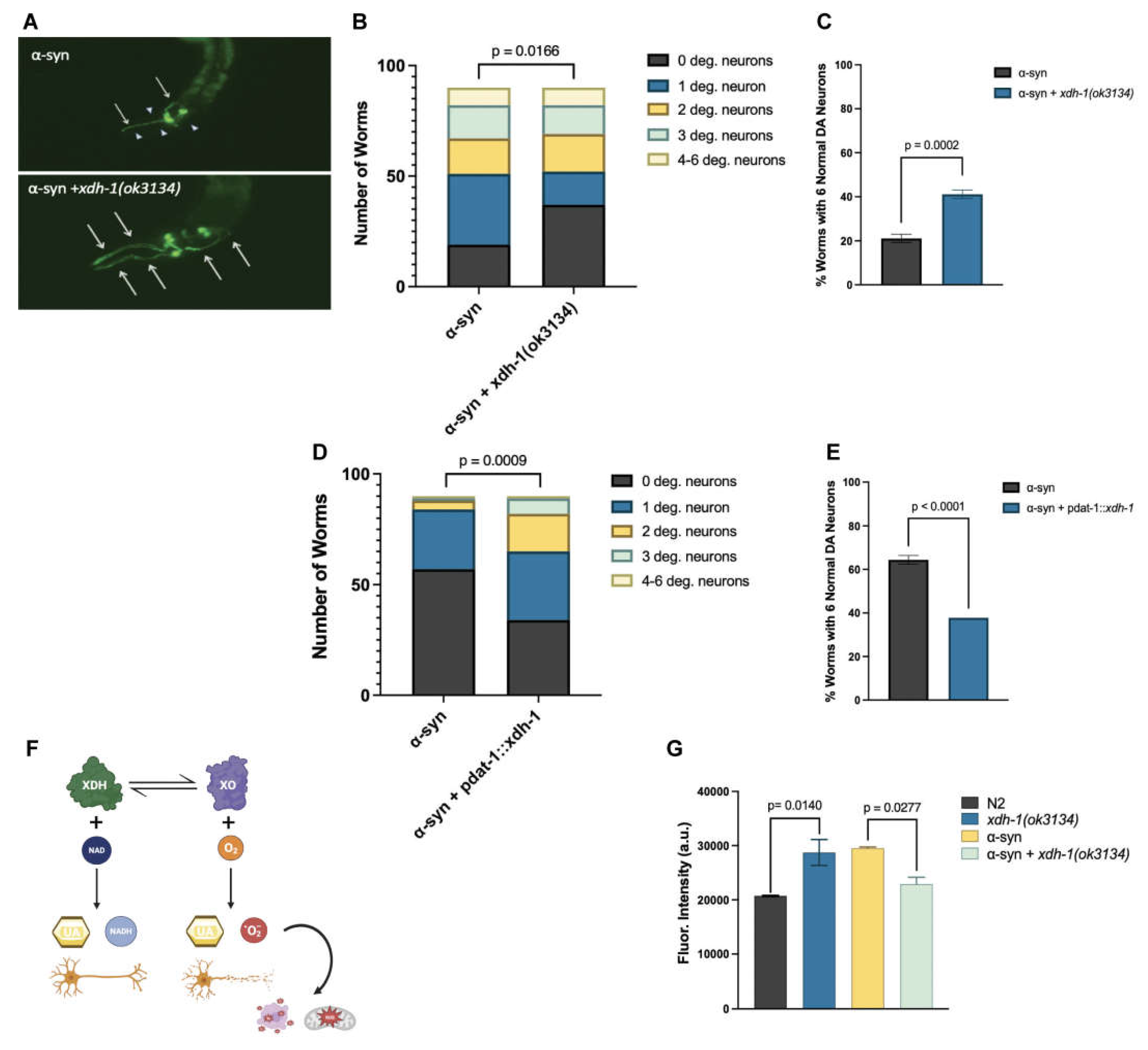
Figure 4.
xdh-1, wht-2 and adr-2 comprise a genetic regulatory network that affects PD pathologies in a tissue-specific manner. (A) Synchronized worms were scored for dopamine neurodegeneration seven days post-hatch. Knockdown of xdh-1 and xdh-1 + adr-2 indicate that adr-2 does not impact xdh-1 activity, while knockdown of wht-2 + xdh-1 indicate that xdh-1 does not regulate wht-2 activity. These experiments were completed via RNAi knockdown in dopaminergic neurons of C. elegans expressing α-syn + GFP under control of the Pdat-1 promoter (three independent experiments, n = 30 per independent groups; N=3; n=30). Significance was obtained using a One-way ANOVA with a Tukey post hoc test; ns >0.05. (B) The same experimental paradigm was performed in worms expressing α-syn and GFP in the body wall muscles. Synchronized two-day old worms were scored for aggregate size and number, which were combined to generate an overall aggregate score. The relation between adr-2, xdh-1 and wht-2 in the body wall match that shown in dopaminergic neurons, implying that wht-2 is the rate-limiting member of this system (three independent experiments, n = 30 per independent groups; N=3; n=30). A One-way ANOVA with a Tukey post hoc analysis was employed, ns >0.05.
Figure 4.
xdh-1, wht-2 and adr-2 comprise a genetic regulatory network that affects PD pathologies in a tissue-specific manner. (A) Synchronized worms were scored for dopamine neurodegeneration seven days post-hatch. Knockdown of xdh-1 and xdh-1 + adr-2 indicate that adr-2 does not impact xdh-1 activity, while knockdown of wht-2 + xdh-1 indicate that xdh-1 does not regulate wht-2 activity. These experiments were completed via RNAi knockdown in dopaminergic neurons of C. elegans expressing α-syn + GFP under control of the Pdat-1 promoter (three independent experiments, n = 30 per independent groups; N=3; n=30). Significance was obtained using a One-way ANOVA with a Tukey post hoc test; ns >0.05. (B) The same experimental paradigm was performed in worms expressing α-syn and GFP in the body wall muscles. Synchronized two-day old worms were scored for aggregate size and number, which were combined to generate an overall aggregate score. The relation between adr-2, xdh-1 and wht-2 in the body wall match that shown in dopaminergic neurons, implying that wht-2 is the rate-limiting member of this system (three independent experiments, n = 30 per independent groups; N=3; n=30). A One-way ANOVA with a Tukey post hoc analysis was employed, ns >0.05.
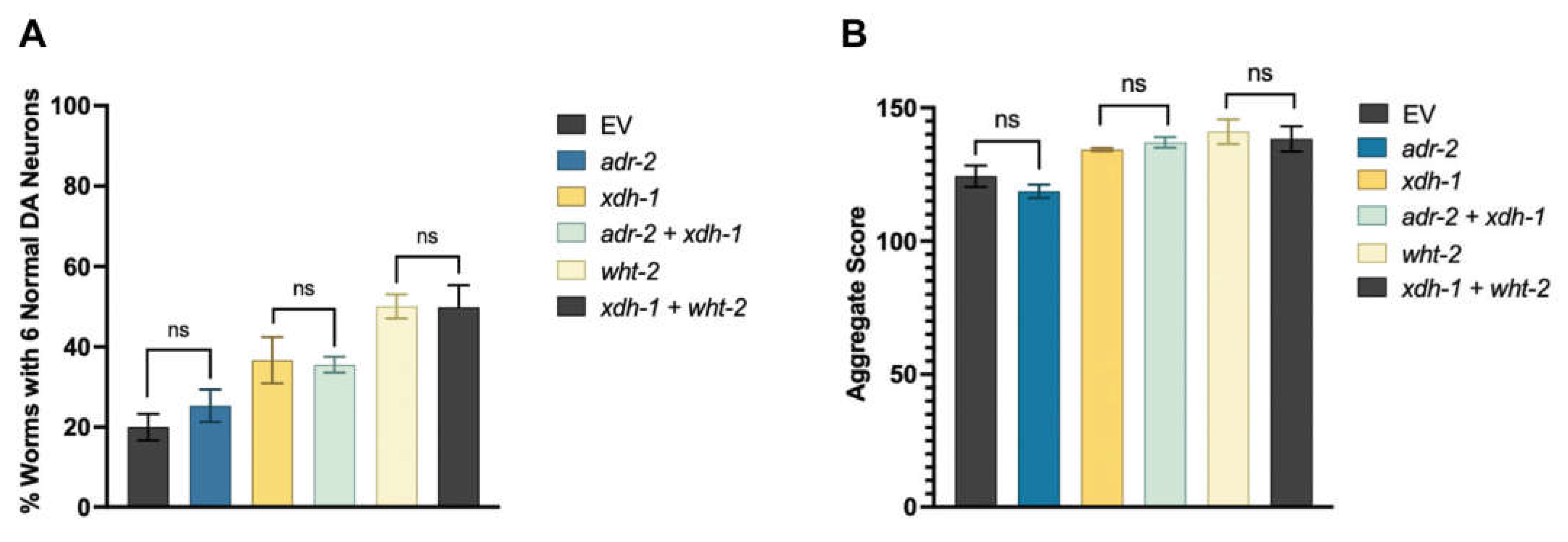
Figure 5.
RNA editing of wht-2 leads to a non-synonymous amino acid substitution that is predicted to alter protein folding. (A) Editing sites in the wht-2 gene. wht-2 consists of 12 exons located at genomic position IV: 11470436 to IV: 11473419 in the C. elegans genome. Two bases in the coding mRNA are edited, with one resulting in an amino acid substitution upon translation. (B) Comparison of the wht-2 RNA and amino acid sequences in the presence and absence of editing at IV: 11470944. Key indicates AlphaFold model prediction confidence. (C) Pairwise alignment between C. elegans wht-2 (top) and human ABCG2 (bottom) DNA sequences. The identified editing site, indicated in red, is located in a conserved region. (D) The complete structure of the unedited WHT-2 protein. Arrow indicates residue 124 where threonine is switched to alanine when RNA is edited, outlined in green. (E-F) Changes in protein structure at residue 124 when WHT-2 is not edited (D) versus edited (E). Hydrogen bonding (shown in green) and protein folding are altered because of editing.
Figure 5.
RNA editing of wht-2 leads to a non-synonymous amino acid substitution that is predicted to alter protein folding. (A) Editing sites in the wht-2 gene. wht-2 consists of 12 exons located at genomic position IV: 11470436 to IV: 11473419 in the C. elegans genome. Two bases in the coding mRNA are edited, with one resulting in an amino acid substitution upon translation. (B) Comparison of the wht-2 RNA and amino acid sequences in the presence and absence of editing at IV: 11470944. Key indicates AlphaFold model prediction confidence. (C) Pairwise alignment between C. elegans wht-2 (top) and human ABCG2 (bottom) DNA sequences. The identified editing site, indicated in red, is located in a conserved region. (D) The complete structure of the unedited WHT-2 protein. Arrow indicates residue 124 where threonine is switched to alanine when RNA is edited, outlined in green. (E-F) Changes in protein structure at residue 124 when WHT-2 is not edited (D) versus edited (E). Hydrogen bonding (shown in green) and protein folding are altered because of editing.
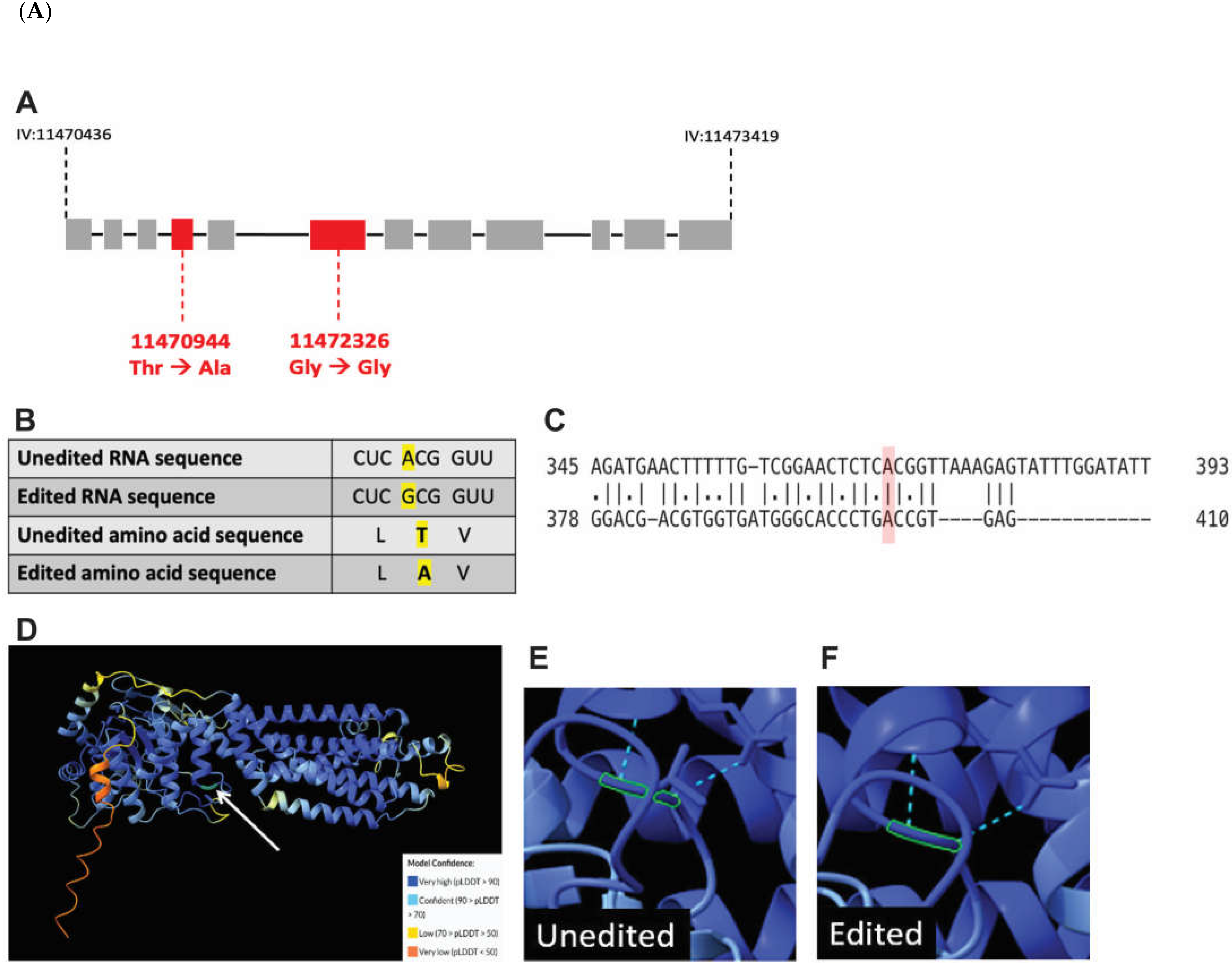
Figure 6.
A proposed model of ADR-2, WHT-2 and XDH-1 activity. In the presence of ADR-2, WHT-2 is edited which allows for optimal export of uric acid, a product of purine catabolism by XDH-1. In the absence of editing by ADR-2, the structure of WHT-2 is altered such that uric acid cannot efficiently be exported out of the cell. To maintain cellular homeostasis, there is a downregulation of xdh-1 transcription. Created with Biorender.com.
Figure 6.
A proposed model of ADR-2, WHT-2 and XDH-1 activity. In the presence of ADR-2, WHT-2 is edited which allows for optimal export of uric acid, a product of purine catabolism by XDH-1. In the absence of editing by ADR-2, the structure of WHT-2 is altered such that uric acid cannot efficiently be exported out of the cell. To maintain cellular homeostasis, there is a downregulation of xdh-1 transcription. Created with Biorender.com.
Table 1.
Summary of crosses completed to generate new strains used in this study.
Table 1.
Summary of crosses completed to generate new strains used in this study.
Strain |
Genotype |
Strains Crossed |
UA455 |
xdh-1(ok3134); baIn11[Pdat-1::α-syn, Pdat-1 ::GFP] |
RB2379 x UA44 |
UA456 |
xdh-1(ok3134); vtIs7 [Pdat-1::GFP] |
RB2379 x BY250 |
UA457 |
adr-2(ok735); vtIs7[Pdat-1::α-syn, Pdat-1 ::GFP] |
RB886 x BY250 |