1. Introduction
The possibility of combining different types of chemical compounds has given rise to the development of new molecules with improved pharmacological characteristics. Among these, peptides, and antimicrobial conjugates, offering expanded molecular targets, reduced toxicity, and the ability to overcome drug resistance when compared to their original substances [
1,
2,
3,
4,
5,
6,
7,
8]. Cell-Penetrating Peptides (CPPs) and Antimicrobial Peptides (AMPs) are two types of peptides that demonstrate not only antimicrobial properties but also anticancer potential [
2,
4,
9,
10,
11,
12,
13,
14,
15,
16]. As previously demonstrated, CPPs like TP10 and its fluoroquinolone conjugates show antibacterial, and anti-leukemic effects minimal generation of ROS and slight impact on mitochondrial functions [
8,
17].
Crucially, leukemic cells frequently display disturbances in the outer lipid membrane, making them highly sensitive to compounds that interfere with membrane integrity [
18,
19,
20,
21,
22,
23,
24,
25]. These lipid disturbances have been identified as a cellular target for hematological malignancies [
26,
27,
28,
29,
30]. Compounds with these properties provide an opportunity for alternative treatment of leukemia patients undergoing HCT [
7]. HCT continues to be highly effective in treating leukemia, even as anti-leukemic therapies evolve [
31,
32,
33,
34,
35,
36]. Nevertheless, in the context of HCT, susceptibility to microbial infections remains a significant factor contributing to morbidity and mortality, with rates ranging from 19% to 44% [
37,
38,
39]. This is a notable problem for both children with acute lymphoid leukemia, and older patients with diverse leukemia types [
40,
41,
42,
43].
For this reason, HCT treatment requires immunosuppressive drugs, increasing the risk of microbial infections. The standard antimicrobials used in this context are Ciprofloxacin (CIP) and Levofloxacin (LVX) [
38,
44,
45,
46]. As a drawback, antibacterial treatment with CIP and LVX induces toxicity [
47,
48,
49]. Meanwhile, it is established that the antibacterial activity of CIP and LVX strongly depends on topoisomerase inhibition [
47,
48,
49]. Our data obtained on fluoroquinolones conjugated to TP10 [
8,
17], as well as chimera peptides composed of Human Neutrophil Peptide (HNP), demonstrate selectivity against microbes and leukemic cells, compared to healthy human cell cultures [
53]. To investigate the efficacy of an AMP other than CPP combined with antibiotics, we selected LfcinB, an AMP with established anticancer activity [
54,
55,
56,
57,
58,
59,
60,
61] and low toxicity [
62,
63].
In this update, expanding on the previously shared findings related to the biological activity of the LfcinB and antibiotic combination [
64], we performed additional MTT tests for compound 4 ((LfcinB covalently conectet to Fluconazole (FLC)) and on HL60 and HEK293 cell lines. Furthermore, we calculated the Bacteriostatic Selectivity Index (B
SI), Fungistatic Selectivity Index (F
SI), and Malignancy Selectivity Index (M
SI). To assess the basal molecular impact of LfcinB and selected conjugates on bacteria, we examined
S. aureus alterations in the asymmetric distribution of phosphatidylserine in the membrane, along with
in vitro bacterial topoisomerase inhibition assays. Furthermore, we expanded our research on potential toxicity by examining intracellular ROS generation in HL60 and HEK293 [
64] and assessing their influence on mitochondrial functions.
Highlighting their leukemic selectivity and antimicrobial activity, along with a minimal impact on ROS generation and mitochondrial function, we have identified an interesting compound, designated as number 3 (disulphide bridge between LfcinB and CIP). This conjugate demonstrates all these properties at an IC
50 of a micromolar concentrations and an EC
50 - Effective Concentration (IC normalized to cell number [
65]) in the nanomolar range. It is worth noting that conjugate 3 serves as an example of a disulfide connection between parental drugs, representing a promising target for enzymatic reduction.
2. Results
2.1. Inhibition of Cancer Cells Proliferation and EC50 of Lfcin and Its Conjugates
To enhance the previously published dataset on the inhibition of cancer cell growth by LfcinB and its conjugates [
61], we conducted additional experiments for conjugate 4 on HL60 and HEK293. The antiproliferative effects of LfcinB and its conjugate 4 were evaluated using the MTT assay, and the calculated IC
50 values are detailed in Supplementary Table ST1. However, the cellular response to compound 4 for HL60 was approximately 65 μM. It is noteworthy that the IC
50 of the leukemic cells was still approximately 2.5 times lower than that observed in healthy HEK293 cells.
When it comes to calculating and comparing EC
50 values for LFcinB and its all conjugates (
Figure 1a) on HL60, BT20, A549, and HEK293 cell lines, conjugates 1 (covalently bounded LfcinB and LVX) and 3 proved to be the most active against cancer cells, with EC
50 ranging from 3-69 nM (Suplementary Table ST2). In contrast, conjugate 4 exhibited an EC
50 of 108 nM in healthy HEK293 cells. Notably, the FLC conjugate, compared to those containing CIP and LVX, showed approximately twice as high EC
50 values on all tested cancer cell lines (Supplementary Table ST2). The most significant growth inhibition was observed for conjugate 3 on HL60, with an EC
50 of 3 nM, while the highest values were recorded for LfcinB (499 nM) and conjugate 3 (409 nM) in HEK293. The most sensitive cell line to the action of LFcinB and all tested conjugates was HL60, with EC
50 ranging from 3-13 nM and the least sensitive turned out to be HEK293, with EC
50 ranging from 108-499 nM (Supplementary Table ST2).
2.2. The Biological Selectivity of LfcinB and its Conjugates
2.2.1. Bacteriostac Selectivity
B
SI values were analyzed concerning bacteria strains: S. epidermidis, B. subtilis, S. aureus, E. coli, P. aeruginosa, B. cereus, as well as cell cultures: leukemia (HL60), breast cancer (BT20), non-small cell lung cancer (A549), and non-cancerous embryonic kidney cells (HEK293), represented by MIC/IC
50 (
Supplementary Table S2) and B
SI indexing illustrated in
Figure 1b.
Figure 1.
Compound Structures and Selectivity Indexing Panels. (a) Chemical structures of LfcinB, antibiotics and conjugates; LfcinB structure was generate by
https://www.rcsb.org/ (accessed on November 2023); Peptide SMILES was generate by
https://www.novoprolabs.com/ (accessed on November 2023); (b) Antimicrobial Selectivity normalized to IC
50 HL60; (c) Malignancy Selectivity normalized to EC
50; Selectivity indexing: Red – none, Yellow – moderate, Light green – good, Deep green – very good; IC
50 - Inhibitory Concentration; EC
50 - Effective Concentration (IC normalized to cell number); Abbreviations:
S. e. - Staphylococcus epidermidis ATCC 12228;
B. s. - Bacillus subtilis ATCC 6633;
S. a. - Staphylococcus aureus ATCC 25923;
Escherichia coli ATCC 25922;
P. a - Pseudomonas aeruginosa ATCC 27853;
B. c - Bacillus cereus PCM 2003;
C. k. - Candida krusei DSM 6128;
C. p. - Candida parapsilosis DSM 5784;
C. a. - Candida albicans ATCC 10231;
C. g. - Candida glabrata DSM 11226;
C. t. - Candida tropicalis CZD 519; For more detailed information please refer to the Supplementary Table ST2.
Figure 1.
Compound Structures and Selectivity Indexing Panels. (a) Chemical structures of LfcinB, antibiotics and conjugates; LfcinB structure was generate by
https://www.rcsb.org/ (accessed on November 2023); Peptide SMILES was generate by
https://www.novoprolabs.com/ (accessed on November 2023); (b) Antimicrobial Selectivity normalized to IC
50 HL60; (c) Malignancy Selectivity normalized to EC
50; Selectivity indexing: Red – none, Yellow – moderate, Light green – good, Deep green – very good; IC
50 - Inhibitory Concentration; EC
50 - Effective Concentration (IC normalized to cell number); Abbreviations:
S. e. - Staphylococcus epidermidis ATCC 12228;
B. s. - Bacillus subtilis ATCC 6633;
S. a. - Staphylococcus aureus ATCC 25923;
Escherichia coli ATCC 25922;
P. a - Pseudomonas aeruginosa ATCC 27853;
B. c - Bacillus cereus PCM 2003;
C. k. - Candida krusei DSM 6128;
C. p. - Candida parapsilosis DSM 5784;
C. a. - Candida albicans ATCC 10231;
C. g. - Candida glabrata DSM 11226;
C. t. - Candida tropicalis CZD 519; For more detailed information please refer to the Supplementary Table ST2.
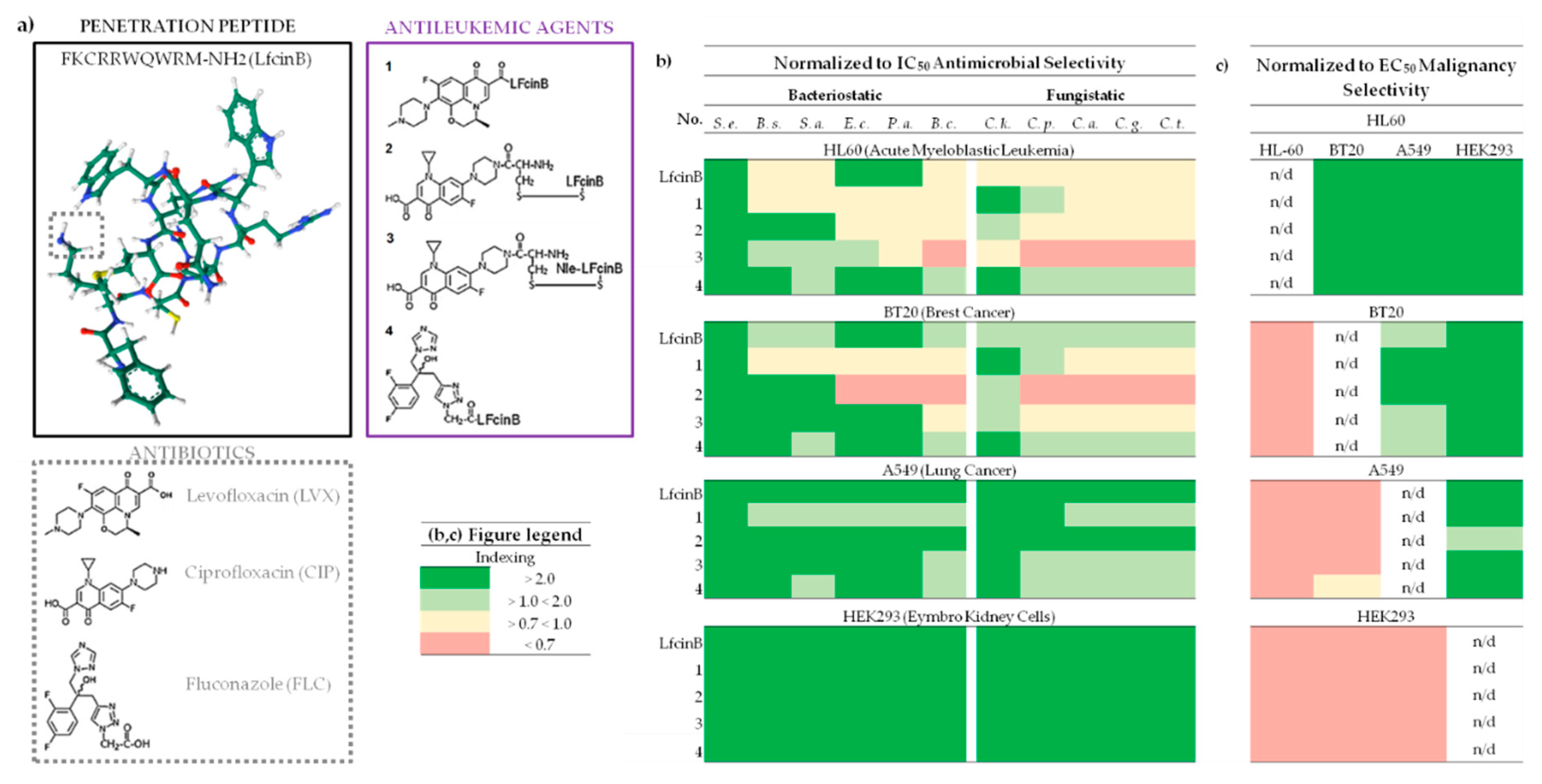
LfcinB and conjugate 3 demonstrated the most favorable B
SI across all tested cell lines and bacterial strains (
Figure 1b). S. epidermidis exhibited highly favorable B
SI, with B
SI >5 and <472, while B. cereus showed the least favorable values with B
SI <0.7 and <16 (
Figure 1b and Supplementary Table ST2). For HEK293, all B
SI calculations for LfcinB and its conjugates indicated high favorability (>2) against all tested strains. Conjugate 3, in particular, achieved the best score against S. epidermidis with a BSI >471, and LfcinB showed a B
SI >179.
The least favorable index was noted for the HL60 cell line, where, apart from the values for S. epidermidis with B
SI ranging from (>6 and <13), the index was varied from unfavorable (B
SI > 0.7), as seen in the case of conjugate 3 on B. cereus, to very good (B
SI >13 and <14), as observed with conjugate 2 (alternative disulfbrige conection betwee peptide and CIP) on S. epidermidis, but mostly were not more around 3, mainly ranging aproximitly 0.7 (
Figure 1b and Supplementary tabble ST2). This outcome exibit that, in HL-60, the bacteriostatic effect is low effitient than cytostatic obtained on leukemic cells.
For BT20 cell line, the B
SI results were similar to HL60 , with the cancer cytostatic effect especially for conjugates 1 and 2, surpassing the bacteriostatic (
Figure 1b). Notably, the difference in the action of conjugates 2 and particulary 3 (connected by disulfide bonds) between HL60 and BT20 for E. coli, P. aeruginosa, B. cereus suggests that such difference in combination might be associated with a more selective cytostatic effect against leukemia (
Figure 1b).
2.2.2. Fungistatic Selectivity
F
SI values were analyzed concerning bacteria strains: C. krusei, C. parapsilosis, C. albicans, C. glabrata, C. tropicalis as well as cell cultures: leukemia (HL60), breast cancer (BT20), non-small cell lung cancer (A549), and non-cancerous embryonic kidney cells (HEK293), represented by MIC/IC
50 (
Supplementary Table S2) and F
SI indexing in
Figure 1b.
Conjugate 4 (containing FLC) and LfcinB exhibited the most desirable F
SI across all tested cell lines and bacterial strains (
Figure 1b). C. krusei showed a favorable F
SI of >7 and <34, while the other three strains demonstrated lower values with F
SI <0.7 and <18 (
Figure 1b and Supplementary Table ST2). Similarly, for HEK293, all F
SI calculations for LfcinB and its conjugates indicated favorability (>2) against all tested strains. Conjugate 3 exibited favorable Fsi, ranging from >15 to <31 for all strains.
The least favorable index was noted for HL60 (
Figure 1b). The index was mostly unfavorable (F
SI > 0.7), or moderate (<0.7 and >1), and very good in the case of C. krusei for compounds 1 and 4, with F
SI values of 2.9 and 2.4, respectively. As for B
SI, the fungistatic effect was lower than the antiproliferative effect on leukemic culture.
The F
SI results for BT20 resembled those for HL-60. The main differences observed across all strains were a more favorable F
SI for BT20 with LfcinB and conjugate 3, while it was less favorable for conjugate 2. Therefore, as in the case of B
SI, the differences in F
SI may suggest a more selective cytostatic effect against leukemic cells (
Figure 1b).
2.2.3. Malignancy Selectivity
To evaluate their effect on leukemia, cancer and non-cancer cells, the Malignancy Selectivity Index was caltulated as M
SI = EC
50 (non-cancerous) / EC
50 (leukemic or cancerous) or EC
50 (leukemic or cancer) / EC
50 (leukemic or cancerous). This data is presented in Supplementary Table ST3 and visualized in
Figure 1c. Most compounds displayed higher M
SI for cancer especially leukemic cells, compared to healthy cells (
Figure 1c, Supplementary Table ST3).
M
SI for all compounds reached their peak for HL60, with a range between >3 and the highest score <136 obtained for compound 3 on HEK293 (refer to Supplementary Table ST3). LfcinB also displayed promising M
SI parameters for HL60, attaining values between >8 and <52. Furthermore, disulfide bridge conjugates 2 and 3 exhibited higher M
SI in HL60 than their counterparts 1 and without the bridge connection (see
Figure 1c). HL60 was the only cell line that exhibited favorable M
SI across all compounds when compared to two other cancer cell lines and the non-cancerous HEK293 cells. Notably, all the compounds demonstrated selectivity towards healthy cells, indicated by negative M
SI (
Figure 1c).
2.3. LfcinB and Its Conjugates Eliminating Bacteria
2.3.1. Switch the Asymmetry in Membrane Phospholipids
Our study incorporates insights from Dwyer's research, emphasizing that bacteria can display essential characteristics of eukaryotic cell death [
66]. In the dot plots shown in
Figure 2a (7AAD vs. Annexin V), there is a notable reduction in the number of events in samples treated with compounds compared to the untreated control over 6 hours. Specifically, when compared to LfcinB, the impact of conjugate 3 (at MIC concentrations) is more pronounced, reflected in a lower number of events from the first hour to six hours. At this point of time, we observe essential disturbances in the asymmetric distribution of phosphatidylserine in the membrane (as indicated by Annexin V level), leading to a loss of integrity (evidenced by 7AAD accumulation) and subsequent cell death. Consequently, the bacteriostatic effect induced by the compound also manifests as bactericidal (
Figure 1b and Figure 2a).
2.3.2. DNA Gyrase and Topoisomerase IV Inhibition
Disruptions in the membrane, triggered by the treatment with compounds, result in a loss of cell integrity, enhance the entry of these compounds into the cell. Our study indicates that topoisomerase IV serves as the primary target for quinolones and their conjugates. We evaluated seven compounds for their inhibitory effects on S. aureus topoisomerases using three assays: gyrase activity, relaxation, and topoisomerase IV activity (
Figure 2b). The LfcinB peptide alone exerted the most significant impact on topoisomerase IV relaxation activity, while compound *5 (chimera CH9 of HNP-1 for more details please refer to [
53]) demonstrated the highest inhibitory effect among tested coumpounds (
Figure 2b). Notably, LfcinB, when conjugated with CIP, exhibited a relatively high inhibitory effect on all enzyme activities, compared to its connection to LVX (
Figure 2b).
2.4. LfcinB and Its Conjugates Exhibit Low Toxicity in Human Cell Lines
To verify whether the observed leukemic-selective cytostatic effect is potentially toxic, we expanded our previous studies [
64] to include the evaluation of intracellular ROS generation by compound 4 (
Figure 2c). Furthermore, we investigated the impact of the selected conjugate on mitochondrial functions (
Figure 2d). The ROS-generating activity of conjugates 4 with FLC was evaluated in HL60 and HEK293 cell lines (Figure 3a). We employed the fluorescent CM-H2DCFDA probe for ROS detection , with H
2O
2 used as a positive control due to its known role as anROS inducer. Incubation with compound 4 (at 2-fold IC
90) resulted in negligible levels of cellular ROS (
Figure 2c). Importantly, these findings align with the data reported for conjugates 1-3, as detailed in our earlier publication [
64]. The obtained evidences strongly suggest that the cytostatic effect is ROS-independent (
Figure 2c). To further investigation, we examined whether the compounds could causemitochondrial dysfunction. To confirm this, we assessed mitochondrial potential through flow cytometry using HL60 cells stained with DIOC6(3) and treated with compound 3 (
Figure 2d). Our analysis showed that the tested conjugate showed a minimal impact on mitochondrial potential, suggesting a low risk of toxicity in this context.
3. Discussion
Antimicrobial Selectivity Provide by Antimicrobial Peptide Combine with Antibiotic
AMPs are small molecules with positive charged molecules with amphipathic structures. They are found widely in animal tissues and serve as a crucial first line of defense against infections. AMPs exhibit a broad spectrum of activity, mainly targeting bacteria, fungi, and viruses [
67], and this phenome extends to LfcinB and its conjugates as well (
Figure 1a, b). The primary mode of action involves the direct disruption of microbial cell membranes, selectively binding to negatively charged phospholipid head groups on bacterial membranes [
68]. According to our findings, this type of effect occurs not only for LfcinB (
Figure 2a). Importantly, AMPs are not attracted to neutral host cell membranes [
66]. This effect might have influenced the low activity of all tested compounds against noncancerous HEK293 cells and their significantly higher activity against cancer and leukemic cells (
Figure 1c).
In addition to membrane disruption, AMPs can impact microbial cells through various mechanisms, including interactions with intracellular targets such as nucleic acids or proteins, as well as the disruption of cellular processes. Fluoroquinolones exert their antimicrobial effect by inhibiting two essential enzymes involved in bacterial DNA synthesis: DNA gyrase and topoisomerase IV [
70]. DNA gyrase introduces negative supercoils in bacterial DNA, facilitating the separation of daughter chromosomes necessary for replication initiation. Topoisomerase IV is responsible for the decatenation process, separating daughter chromosomes at the end of replication [
71,
72]. Fluoroquinolones form complexes with either DNA gyrase or topoisomerase IV bound to bacterial DNA, causing conformational changes that deactivate these enzymes. This interference impedes the progression of the replication fork and bacterial DNA synthesis, ultimately leading to rapid bacterial cell death [
70].
Figure 2b illustrates the inhibition the DNA-gyrase in Gram-negative and DNA-topoisomerase IV in Gram-positive bacteria in an in vitro assay induced by fluoroquinolones.
Antileukemic Selectivity and Low Potential Toxicity
Leukemic cells, sensitive to compounds disrupting the outer lipid membrane, represent a target for hematological malignancies [
73]. As we previously demonstrated with CPP, TP10, and its conjugates with CIP and LVX, these compounds exhibited selective and low toxicity toward leukemic cells [
17]. Consequently, certain AMPs may also be active against these cell types. Our research revealed that LfcinB and its conjugates exhibit selectivity towards leukemic cells, antimicrobial activity, and minimal impact on ROS generation and mitochondrial function (
Figure 1 and
Figure 2c,d). These AMPs, when combinated with antimicrobial agents, demonstrate potential for effectively targeting leukemia cells and managing infections during HCT.
4. Materials and Methods
4.1. Drugs
Following compounds were purchased from Sigma-Aldrich (USA): 7-aminoactinomycin (Cat. A9400), CCCP (Cat. 215911), ciprofloxacin (Cat. 17850), levofloxacin (Cat. 1362103), DMSO (Cat. D8418), LfcinB and conjugates 1-4 were synthesized using the method detailed in our prior work [Ptaszyńska et al., 2019]. Compound *5 was synthesized according to the procedure described in [Ptaszyńska et al., 2018]. All drugs were dissolved in DMSO at concentrations ranging from 2 to 10 mM and stored at −20 °C. 7-AAD was dissolved in MeOH:H2O (4:6) and stored at 4 °C.
4.2. Cell Cultures
HL-60, CEM and A-549 were acquired from ATCC. HL60 was cultured in RPMI-1640 medium (Cat. R5158, Merck) and HEK293 Eagle’s Minimum Essential Medium (Cat. M5775, Merck). Both lines were supplemented with 10% FBS (Cat. F7524, Sigma-Aldrich), 2 mM L-glutamine (Cat. G8540, Sigma-Aldrich), and the antibiotics penicillin (100 U/mL) and streptomycin (100 mg/mL) at 37 °C in a humidified atmosphere of 5% CO2 and 95% air. Cell lines were routinely screened for mycoplasma contamination. Cell density was measured using Coulter Z2 (Beckman, USA) equipped with 100 µm aperture or using the flow cytometer Guava EasyCyte 8HT (Merck-Millipore, USA).
4.3. Determination of Drug Cytotoxicity
As mentioned in [
61], we followed detailed procedures, including the composition of media and growth conditions, for the application of conjugate 4 on both HL60 and HEK293 in the MTT drug sensitivity assay. The initial cellular density for cultures seeded in a 96-well plate was 5000 cells/well for HL60 and 1500 cells/well for HEK293.
4.4. Switch of S. Aureus Asymmetric Phosphatidylserine Location
Bacterial cultures with an OD200, as utilized in bacteriostatic experiments (Ptaszyńska et al., 2019), were plated on Petri dishes. Compounds were added at the MIC concentration to the test sample, while no compound was added to the negative control. After an appropriate incubation period (45 min, 2.45 min, and 5.45 min), samples were collected and promptly washed with PBS. The pellet was then suspended in 100 μL of annexin binding buffer (BioSource International), which included 0.25 μL of 7-AAD (7-aminoactinomycin D, 1 mg/mL, Sigma), and 1 μL of Annexin-FITC (ThermoFisher). Following the addition of 400 μL of annexin binding buffer, the samples were left in the dark for 15 min. Subsequently, the samples were placed on ice and immediately read by Guava easyCyte (Merck-Millipore, Burlington, MA, USA). Flow results were analyzed with the free online software
https://floreada.io/ (accessed in November 2023; the last update was carried out in June 2023).
4.5. Inhibition of S. Aureus Gyrase and Topoisomerase IV Activities
Inhibitory concentrations for three gyrase and topoisomerase IV activities were assessed: gyrase supercoiling, TOPO IV relaxation and TOPO IV decatenation. For this, commercially available kits were used (S. aureus gyrase supercoiling assay kit, S. aureus topoisomerase IV relaxation assay kit and topoisomerase IV decatenation kit all purchased from Inspiralis (UK)). The procedure was carried out according to the manufacturer’s instructions. The substrates for the reactions were: 500 ng of the relaxed pBR322 plasmid, 500 ng of supercoiled pBR322 or 200 ng of kinetoplast DNA (kDNA) from Crithidia fasciculate, respectively. The studied compounds were dissolved and diluted in dd H2O at the experimentally selected concentrations (gyrase: 2000-250 μM for CIP, 1000-50 μM – LVX, 500-50 μM conjugates, topoisomerase: 500-5 μM CIP/LVX, 250-10 μM – conjugates). Appropriate controls were included (with or without the enzyme or without the addition of the tested compound). After the addition of the indicated amount of the enzyme, the reaction proceeded at 37 °C for 30 min. Loading buffer was used for reaction termination. The studied compounds were extracted from the reaction mixtures with 30 µL of chloroform/isoamyl alcohol solution (24:1; v/v). After centrifugation (3 min, 20,000 g), the upper aqueous phase was loaded on 1% agarose gels and run at 90 V for 4 hours in TBE buffer (90 mM Tris-base, 70 mM boric acid, 1 mM EDTA, pH 8). Gels were stained with 1 μg/mL ethidium bromide (Sigma, USA) for 15 min to visualize DNA and unbound ethidium bromide was removed by washing gel in dd H2O for 15 min.
4.6. Intracellular ROS Generation
Cell lines were cultured as mentioned above and seeded in Petri dishes (Falcon, USA) in the amount of 25x103 per Φ35 dish. HEK293 were allowed to attach overnight. ROS generation potential was tested for selected compounds after 0.25, 0.5, 1, 3, 6 and 24 h of incubation. CM-H2DCFDA molecular probe (Thermo Fischer Scientific, Waltham, USA) at final concentration 1 µM was added 15 min before the analysis. After staining HEK293 cells were trypsinised, harvested and suspended in fresh media. 7AAD (Cat. A9400, Sigma Aldrich) was added just before analysis. Analyses were carried out with Guava easyCyte flow cytometer (Merck-Millipore, USA). Flow cytometry data was processed with Flowing Software 2.5.1 (Turku Bioscience, Finland).
4.7. Investigation of Mitochondrial Potential
HL60 was incubated with 100 nM 3,3`-dihexyloxacarbocyanine iodide (DiOC6(3)) (Cat. D273, Thermo Fischer Scientific). Cells were stained under culture conditions for 15 min, washed with prewarmed PBS, and performed Guava easyCyte flow cytometer (Merck-Millipore, USA). Results of flow cytometry were analyzed with Flowing Software 2.5.1 (Turku Bioscience, Finland).
5. Conclusions
Combining PPs with antimicrobial agents exhibit potential for effectively targeting leukemia cells and addressing infections during HCT.
Supplementary Materials
The following supporting information can be downloaded at the website of this paper posted on Preprints.org.
Author Contributions
Conceptualization, J.J.L., K.G., M.H., A.S., N.P., A.Ł., B.P., J.J.B. and K.R.; methodology, J.J.L., K.G., A.S. and M.H.; software, J.J.L, K.G. and M.H.; validation, J.J.L., K.G., A.S. and M.H.; formal analysis, J.J.B. and K.R.; investigation, J.J.L, K.G., M.H., A.S., J.J.B. and K.R.; resources, K.R.; data curation, J.J.L., K.G. and M.H.; writing—original draft preparation, J.J.L., K.G., M.H., A.S., N.P., A.Ł., B.P., N.M., A.G.D., D.D., J.J.B. and K.R.; visualization, J.J.L. and K.G.; supervision, J.J.L. and K.R.; project administration, J.J.L. and K.R.; funding acquisition, K.R.
Funding
This work was supported by the National Science Centre (NCN, Poland) under grant no. UMO-2016/21/B/ST5/00101
Institutional Review Board Statement
Not applicable.
Informed Consent Statement
Not applicable.
Data Availability Statement
The authors declare that all the imaging and biological data supporting the findings of this study are available within the paper and its Supplemental Data.
Conflicts of Interest
The authors declare no conflict of interest.
References
- Duan, Z., Chen, C., Qin, J., Liu, Q., Wang, Q., Xu, X., & Wang, J. (2017). Cell-penetrating peptide conjugates to enhance the antitumor effect of paclitaxel on drug-resistant lung cancer. Drug delivery, 24(1), 752–764. [CrossRef]
- Felício, M. R., Silva, O. N., Gonçalves, S., Santos, N. C., & Franco, O. L. (2017). Peptides with DualAntimicrobial and Anticancer Activities. Frontiers in chemistry, 5, 5. [CrossRef]
- Ruczyński, J., Rusiecka, I., Turecka, K., Kozłowska, A., Alenowicz, M., Gągało, I., Kawiak, A., Rekowski, P., Waleron, K., & Kocić, I. (2019). Transportan 10 improves the pharmacokinetics and pharmacodynamics of vancomycin. Scientific reports, 9(1), 3247. [CrossRef]
- Tornesello, A. L., Borrelli, A., Buonaguro, L., Buonaguro, F. M., & Tornesello, M. L. (2020). Antimicrobial Peptides as Anticancer Agents: Functional Properties and Biological Activities. Molecules, 25(12), 2850. [CrossRef]
- Reissmann, S., & Filatova, M. P. (2021). New generation of cell-penetrating peptides: Functionality and potential clinical application. Journal of peptide science: an official publication of the European Peptide Society, 27(5), e3300. [CrossRef]
- Xie J, Bi Y, Zhang H, Dong S, Teng L, Lee RJ, Yang Z. Cell-Penetrating Peptides in Diagnosis and Treatment of Human Diseases: From Preclinical Research to Clinical Application. Front Pharmacol. 2020 May 20;11:697. [CrossRef]
- Zeiders, S. M., & Chmielewski, J. (2021). Antibiotic-cell-penetrating peptide conjugates targeting challenging drug-resistant and intracellular pathogenic bacteria. Chemical biology & drug design, 98(5), 762–778. [CrossRef]
- Lica, J. J., Heldt, M., Wieczór, M., Chodnicki, P., Ptaszyńska, N., Maciejewska, N., Łęgowska, A., Brankiewicz, W., Gucwa, K., Stupak, A., Pradhan, B., Gitlin-Domagalska, A., Dębowski, D., Milewski, S., Bieniaszewska, M., Grabe, G. J., Hellmann, A., & Rolka, K. (2023). Dual-Activity Fluoroquinolone-Transportan 10 Conjugates offer alternative Leukemia therapy during Hematopoietic Cell Transplantation. Molecular pharmacology, MOLPHARM-AR-2023-000735. Advance online publication. [CrossRef]
- Rusiecka, I., Jarosław, R., Magdalena, A., Piotr, R., & Ivan, K. (2016). Transportan 10 improves the anticancer activity of cisplatin. Naunyn-Schmiedeberg's archives of pharmacology, 389(5), 485–497. [CrossRef]
- Feni, L., & Neundorf, I. (2017). The Current Role of Cell-Penetrating Peptides in Cancer Therapy. Advances in experimental medicine and biology, 1030, 279–295. [CrossRef]
- Zhang, F., Yang, D., Jiang, S., Wu, L., Qin, L., He, H., & Zhang, P. (2018). Current Delivery Strategies to Improve the Target of Cell Penetrating Peptides Used for Tumor-Related Therapeutics. Current pharmaceutical design, 24(5), 541–548. [CrossRef]
- Habault, J., & Poyet, J. L. (2019). Recent Advances in Cell Penetrating Peptide-Based Anticancer Therapies. Molecules, 24(5), 927. [CrossRef]
- Burdukiewicz, M., Sidorczuk, K., Rafacz, D., Pietluch, F., Bąkała, M., Słowik, J., & Gagat, P. (2020). CancerGram: An Effective Classifier for Differentiating Anticancer from Antimicrobial Peptides. Pharmaceutics, 12(11), 1045. [CrossRef]
- Zorko, M., Jones, S., & Langel, Ü. (2022). Cell-penetrating peptides in protein mimicry and cancer therapeutics. Advanced drug delivery reviews, 180, 114044. [CrossRef]
- Rusiecka, I., Gągało, I., & Kocić, I. (2022). Cell-penetrating peptides improve pharmacokinetics and pharmacodynamics of anticancer drugs. Tissue barriers, 10(1), 1965418. [CrossRef]
- Zhou, M., Zou, X., Cheng, K., Zhong, S., Su, Y., Wu, T., Tao, Y., Cong, L., Yan, B., & Jiang, Y. (2022). The role of cell-penetrating peptides in potential anti-cancer therapy. Clinical and translational medicine, 12(5), e822. [CrossRef]
- Ptaszyńska, N., Gucwa, K., Olkiewicz, K., Heldt, M., Serocki, M., Stupak, A., Martynow, D., Dębowski, D., Gitlin-Domagalska, A., Lica, J., Łęgowska, A., Milewski, S., & Rolka, K. (2020). Conjugates of Ciprofloxacin and Levofloxacin with Cell-Penetrating Peptide Exhibit Antifungal Activity and Mammalian Cytotoxicity. International journal of molecular sciences, 21(13), 4696. [CrossRef]
- Klock, J. C., & Pieprzyk, J. K. (1979). Cholesterol, phospholipids, and fatty acids of normal immature neutrophils: comparison with acute myeloblastic leukaemia cells and normal neutrophils. Journal of lipid research, 20(7), 908–911.
- Sánchez-Martín, C. C., Dávalos, A., Martín-Sánchez, C., de la Peña, G., Fernández-Hernando, C., & Lasunción, M. A. (2007). Cholesterol starvation induces differentiation of human leukemia HL60 cells. Cancer research, 67(7), 3379–3386. [CrossRef]
- Nirmalanandhan, V. S., Hurren, R., Cameron, W. D., Gronda, M., Shamas-Din, A., You, L., Minden, M. D., Rocheleau, J. V., & Schimmer, A. D. (2015). Increased pressure alters plasma membrane dynamics and renders acute myeloid leukemia cells resistant to daunorubicin. Haematologica, 100(10), e406–e408. [CrossRef]
- Morel, S., Leahy, J., Fournier, M., Lamarche, B., Garofalo, C., Grimard, G., Poulain, F., Delvin, E., Laverdière, C., Krajinovic, M., Drouin, S., Sinnett, D., Marcil, V., & Levy, E. (2017). Lipid and lipoprotein abnormalities in acute lymphoblastic leukemia survivors. Journal of lipid research, 58(5), 982–993. [CrossRef]
- Sun, L., Shi, Y., Wang, G., Wang, X., Zeng, S., Dunn, S. E., Fairn, G. D., Li, Y. J., & Spaner, D. E. (2018). PPAR-delta modulates membrane cholesterol and cytokine signaling in malignant B cells. Leukemia, 32(1), 184–193. [CrossRef]
- Zhao, L., Zhan, H., Jiang, X., Li, Y., & Zeng, H. (2019). The role of cholesterol metabolism in leukemia. Blood science (Baltimore, Md.), 1(1), 44–49. [CrossRef]
- Giacomini, I., Gianfanti, F., Desbats, M. A., Orso, G., Berretta, M., Prayer-Galetti, T., Ragazzi, E., & Cocetta, V. (2021). Cholesterol Metabolic Reprogramming in Cancer and Its Pharmacological Modulation as Therapeutic Strategy. Frontiers in oncology, 11, 682911. [CrossRef]
- Mehta, A., Ratre, Y. K., Soni, V. K., Shukla, D., Sonkar, S. C., Kumar, A., & Vishvakarma, N. K. (2023). Orchestral role of lipid metabolic reprogramming in T-cell malignancy. Frontiers in oncology, 13, 1122789. [CrossRef]
- Li, H. Y., Appelbaum, F. R., Willman, C. L., Zager, R. A., & Banker, D. E. (2003). Cholesterol-modulating agents kill acute myeloid leukaemia cells and sensitize them to therapeutics by blocking adaptive cholesterol responses. Blood, 101(9), 3628–3634. [CrossRef]
- Hilchie, A. L., Vale, R., Zemlak, T. S., & Hoskin, D. W. (2013). Generation of a hematologic malignancy-selective membranolytic peptide from the antimicrobial core (RRWQWR) of bovine lactoferricin. Experimental and molecular pathology, 95(2), 192–198. [CrossRef]
- Li, K., Lv, X. X., Hua, F., Lin, H., Sun, W., Cao, W. B., Fu, X. M., Xie, J., Yu, J. J., Li, Z., Liu, H., Han, M. Z., & Hu, Z. W. (2014). Targeting acute myeloid leukemia with a proapoptotic peptide conjugated to a Toll-like receptor 2-mediated cell-penetrating peptide. International journal of cancer, 134(3), 692–702. [CrossRef]
- Maja, M., & Tyteca, D. (2022). Alteration of cholesterol distribution at the plasma membrane of cancer cells: From evidence to pathophysiological implication and promising therapy strategy. Frontiers in physiology, 13, 999883. [CrossRef]
- Brendolan, A., & Russo, V. (2022). Targeting cholesterol homeostasis in hematopoietic malignancies. Blood, 139(2), 165–176. [CrossRef]
- Slavin, S., Nagler, A., Naparstek, E., Kapelushnik, Y., Aker, M., Cividalli, G., Varadi, G., Kirschbaum, M., Ackerstein, A., Samuel, S., Amar, A., Brautbar, C., Ben-Tal, O., Eldor, A., & Or, R. (1998). Nonmyeloablative stem cell transplantation and cell therapy as an alternative to conventional bone marrow transplantation with lethal cytoreduction for the treatment of malignant and nonmalignant hematologic diseases. Blood, 91(3), 756–763.
- Casper, J., Holowiecki, J., Trenschel, R., Wandt, H., Schaefer-Eckart, K., Ruutu, T., Volin, L., Einsele, H., Stuhler, G., Uharek, L., Blau, I., Bornhaeuser, M., Zander, A. R., Larsson, K., Markiewicz, M., Giebel, S., Kruzel, T., Mylius, H. A., Baumgart, J., Pichlmeier, U., … Beelen, D. W. (2012). Allogeneic hematopoietic SCT in patients with AML following treosulfan/fludarabine conditioning. Bone marrow transplantation, 47(9), 1171–1177. [CrossRef]
- Grossman, J., Cuellar-Rodriguez, J., Gea-Banacloche, J., Zerbe, C., Calvo, K., Hughes, T., Hakim, F., Cole, K., Parta, M., Freeman, A., Holland, S. M., & Hickstein, D. D. (2014). Nonmyeloablative allogeneic hematopoietic stem cell transplantation for GATA2 deficiency. Biology of blood and marrow transplantation : journal of the American Society for Blood and Marrow Transplantation, 20(12), 1940–1948. [CrossRef]
- Cornelissen, J. J., & Blaise, D. (2016). Hematopoietic stem cell transplantation for patients with AML in first complete remission. Blood, 127(1), 62–70. [CrossRef]
- Dandoy, C. E., Ardura, M. I., Papanicolaou, G. A., & Auletta, J. J. (2017). Bacterial bloodstream infections in the allogeneic hematopoietic cell transplant patient: new considerations for a persistent nemesis. Bone marrow transplantation, 52(8), 1091–1106. [CrossRef]
- Zhou, D., Xie, T., Chen, S., Ling, Y., Xu, Y., Chen, B., Ouyang, J., & Yang, Y. (2020). An unusual hematopoietic stem cell transplantation for donor acute lymphoblastic leukemia: a case report. BMC cancer, 20(1), 195. [CrossRef]
- Yeh, S. P., Hsueh, E. J., Yu, M. S., Wu, H., & Wang, Y. C. (1999). Oral ciprofloxacin as antibacterial prophylaxis after allogeneic bone marrow transplantation: a reappraisal. Bone marrow transplantation, 24(11), 1207–1211. [CrossRef]
- Wang, C. H., Chang, F. Y., Chao, T. Y., Kao, W. Y., Ho, C. L., Chen, Y. C., Dai, M. S., Chang, P. Y., Wu, Y. Y., & Lin, J. C. (2018). Characteristics comparisons of bacteremia in allogeneic and autologous hematopoietic stem cell-transplant recipients with levofloxacin prophylaxis and influence on resistant bacteria emergence. Journal of microbiology, immunology, and infection, 51(1), 123–131. [CrossRef]
- Chen, L., Zheng, Y., Yu, K., Chen, S., Wang, W., Gale, R. P., Liu, Z. X., & Liang, Y. (2022). Changing causes of death in persons with haematological cancers 1975-2016. Leukemia, 36(7), 1850–1860. [CrossRef]
- Safdar, A., & Armstrong, D. (2011). Infections in patients with hematologic neoplasms and hematopoietic stem cell transplantation: neutropenia, humoral, and splenic defects. Clinical infectious diseases : an official publication of the Infectious Diseases Society of America, 53(8), 798–806. [CrossRef]
- O'Connor, D., Bate, J., Wade, R., Clack, R., Dhir, S., Hough, R., Vora, A., Goulden, N., & Samarasinghe, S. (2014). Infection-related mortality in children with acute lymphoblastic leukemia: an analysis of infectious deaths on UKALL2003. Blood, 124(7), 1056–1061. [CrossRef]
- Sezgin Evim, M., Tüfekçi, Ö., Baytan, B., Ören, H., Çelebi, S., Ener, B., Üstün Elmas, K., Yılmaz, Ş., Erdem, M., Hacımustafaoğlu, M. K., & Güneş, A. M. (2022). Invasive Fungal Infections in Children with Leukemia: Clinical Features and Prognosis. Turkish journal of haematology : official journal of Turkish Society of Haematology, 39(2), 94–102. [CrossRef]
- Peterson, S. L., Priddy, E., Dong, B. Y., Ghazi, C. A., & Dinglasan, L. A. V. (2019). Evaluation of Infection Risk Factors in Adult Hematologic Malignancy Patients. Biology of Blood and Marrow Transplantation, 25(3), S347. [CrossRef]
- Poutsiaka, D. D., Price, L. L., Ucuzian, A., Chan, G. W., Miller, K. B., & Snydman, D. R. (2007). Blood stream infection after hematopoietic stem cell transplantation is associated with increased mortality. Bone marrow transplantation, 40(1), 63–70. [CrossRef]
- Patel, B., Noda, A., Godbout, E., Stevens, M., & Noda, C. (2020). Levofloxacin for Antibacterial Prophylaxis in Pediatric Patients With Acute Myeloid Leukaemia or Undergoing Hematopoietic Stem Cell Transplantation. The journal of pediatric pharmacology and therapeutics : JPPT : the official journal of PPAG, 25(7), 629–635. [CrossRef]
- Gardner, J. C., Courter, J. D., Dandoy, C. E., Davies, S. M., & Teusink-Cross, A. (2022). Safety and Efficacy of Prophylactic Levofloxacin in Pediatric and Adult Hematopoietic Stem Cell Transplantation Patients. Transplantation and cellular therapy, 28(3), 167.e1–167.e5. [CrossRef]
- Mattappalil, A., & Mergenhagen, K. A. (2014). Neurotoxicity with antimicrobials in the elderly: a review. Clinical therapeutics, 36(11), 1489–1511.e4. [CrossRef]
- Matsubara, R., Kibe, T., & Nomura, T. (2016). Crystalline nephropathy caused by tosufloxacin. Pediatrics international : official journal of the Japan Pediatric Society, 58(11), 1219–1221. [CrossRef]
- Michalak, K., Sobolewska-Włodarczyk, A., Włodarczyk, M., Sobolewska, J., Woźniak, P., & Sobolewski, B. (2017). Treatment of the Fluoroquinolone-Associated Disability: The Pathobiochemical Implications. Oxidative medicine and cellular longevity, 2017, 8023935. [CrossRef]
- Hooper D. C. (2001). Mechanisms of action of antimicrobials: focus on fluoroquinolones. Clinical infectious diseases : an official publication of the Infectious Diseases Society of America, 32 Suppl 1, S9–S15. [CrossRef]
- Blondeau J. M. (2004). Fluoroquinolones: mechanism of action, classification, and development of resistance. Survey of ophthalmology, 49 Suppl 2, S73–S78. [CrossRef]
- Redgrave, L. S., Sutton, S. B., Webber, M. A., & Piddock, L. J. (2014). Fluoroquinolone resistance: mechanisms, impact on bacteria, and role in evolutionary success. Trends in microbiology, 22(8), 438–445. [CrossRef]
- Ptaszyńska, N., Gucwa, K., Łęgowska, A., Dębowski, D., Gitlin-Domagalska, A., Lica, J., Heldt, M., Martynow, D., Olszewski, M., Milewski, S., Ng, T. B., & Rolka, K. (2018). Antimicrobial Activity of Chimera Peptides Composed of Human Neutrophil Peptide 1 (HNP-1) Truncated Analogues and Bovine Lactoferrampin. Bioconjugate chemistry, 29(9), 3060–3071. [CrossRef]
- Duarte, D. C., Nicolau, A., Teixeira, J. A., & Rodrigues, L. R. (2011). The effect of bovine milk lactoferrin on human breast cancer cell lines. Journal of dairy science, 94(1), 66–76. [CrossRef]
- Arias, M., Hilchie, A. L., Haney, E. F., Bolscher, J. G., Hyndman, M. E., Hancock, R. E., & Vogel, H. J. (2017). Anticancer activities of bovine and human lactoferricin-derived peptides. Biochemistry and cell biology = Biochimie et biologie cellulaire, 95(1), 91–98. [CrossRef]
- Chea, C., Haing, S., Miyauchi, M., Shrestha, M., Imanaka, H., & Takata, T. (2019). Molecular mechanisms underlying the inhibitory effects of bovine lactoferrin on osteosarcoma. Biochemical and biophysical research communications, 508(3), 946–952. [CrossRef]
- Ramírez-Sánchez, D. A., Arredondo-Beltrán, I. G., Canizalez-Roman, A., Flores-Villaseñor, H., Nazmi, K., Bolscher, J. G. M., & León-Sicairos, N. (2021). Bovine lactoferrin and lactoferrin peptides affect endometrial and cervical cancer cell lines. Biochemistry and cell biology = Biochimie et biologie cellulaire, 99(1), 149–158. [CrossRef]
- Rocha, V. P., Campos, S. P. C., Barros, C. A., Trindade, P., Souza, L. R. Q., Silva, T. G., Gimba, E. R. P., Teodoro, A. J., & Gonçalves, R. B. (2022). Bovine Lactoferrin Induces Cell Death in Human Prostate Cancer Cells. Oxidative medicine and cellular longevity, 2022, 2187696. [CrossRef]
- Chea, C., Miyauchi, M., Inubushi, T., Okamoto, K., Haing, S., & Takata, T. (2023). Molecular Mechanisms of Inhibitory Effects of Bovine Lactoferrin on Invasion of Oral Squamous Cell Carcinoma. Pharmaceutics, 15(2), 562. [CrossRef]
- Arredondo-Beltrán, I. G., Ramírez-Sánchez, D. A., Zazueta-García, J. R., Canizalez-Roman, A., Angulo-Zamudio, U. A., Velazquez-Roman, J. A., Bolscher, J. G. M., Nazmi, K., & León-Sicairos, N. (2023). Antitumor activity of bovine lactoferrin and its derived peptides against HepG2 liver cancer cells and Jurkat leukemia cells. Biometals : an international journal on the role of metal ions in biology, biochemistry, and medicine, 36(3), 639–655. [CrossRef]
- Ghaly, G., Tallima, H., Dabbish, E., Badr ElDin, N., Abd El-Rahman, M. K., Ibrahim, M. A. A., & Shoeib, T. (2023). Anti-Cancer Peptides: Status and Future Prospects. Molecules (Basel, Switzerland), 28(3), 1148. [CrossRef]
- Yamauchi, K., Toida, T., Nishimura, S., Nagano, E., Kusuoka, O., Teraguchi, S., Hayasawa, H., Shimamura, S., & Tomita, M. (2000). 13-Week oral repeated administration toxicity study of bovine lactoferrin in rats. Food and chemical toxicology : an international journal published for the British Industrial Biological Research Association, 38(6), 503–512. [CrossRef]
- Hayes, T. G., Falchook, G. F., Varadhachary, G. R., Smith, D. P., Davis, L. D., Dhingra, H. M., Hayes, B. P., & Varadhachary, A. (2006). Phase I trial of oral talactoferrin alfa in refractory solid tumors. Investigational new drugs, 24(3), 233–240. [CrossRef]
- Ptaszyńska, N., Olkiewicz, K., Okońska, J., Gucwa, K., Łęgowska, A., Gitlin-Domagalska, A., Dębowski, D., Lica, J., Heldt, M., Milewski, S., Ng, T. B., & Rolka, K. (2019). Peptide conjugates of lactoferricin analogues and antimicrobials-Design, chemical synthesis, and evaluation of antimicrobial activity and mammalian cytotoxicity. Peptides, 117, 170079. [CrossRef]
- Lica, J. J., Wieczór, M., Grabe, G. J., Heldt, M., Jancz, M., Misiak, M., Gucwa, K., Brankiewicz, W., Maciejewska, N., Stupak, A., Bagiński, M., Rolka, K., Hellmann, A., & Składanowski, A. (2021). Effective Drug Concentration and Selectivity Depends on Fraction of Primitive Cells. International journal of molecular sciences, 22(9), 4931. [CrossRef]
- Dwyer DJ, Collins JJ, Walker GC. Unraveling the physiological complexities of antibiotic lethality. Annu Rev Pharmacol Toxicol. 2015; 55:313-32. doi: 10.1146/annurev-pharmtox-010814-124712. Epub 2014 Sep 10. PMID: 25251995. [CrossRef]
- Xia X, Cheng L, Zhang S, Wang L, Hu J. The role of natural antimicrobial peptides during infection and chronic inflammation. Antonie Van Leeuwenhoek. 2018 Jan;111(1):5-26. doi: 10.1007/s10482-017-0929-0. Epub 2017 Aug 30. PMID: 28856473. [CrossRef]
- Hilchie, A. L., Wuerth, K., & Hancock, R. E. (2013). Immune modulation by multifaceted cationic host defense (antimicrobial) peptides. Nature chemical biology, 9(12), 761–768. [CrossRef]
- Pirtskhalava M, Vishnepolsky B, Grigolava M, Managadze G. Physicochemical Features and Peculiarities of Interaction of AMP with the Membrane. Pharmaceuticals (Basel). 2021 May 17;14(5):471. doi: 10.3390/ph14050471. PMID: 34067510; PMCID: PMC8156082.
- Hooper DC, Jacoby GA. Topoisomerase Inhibitors: Fluoroquinolone Mechanisms of Action and Resistance. Cold Spring Harb Perspect Med. 2016 Sep 1;6(9):a025320. doi: 10.1101/cshperspect.a025320. PMID: 27449972; PMCID: PMC5008060. [CrossRef]
- Gubaev A, Klostermeier D. The mechanism of negative DNA supercoiling: a cascade of DNA-induced conformational changes prepares gyrase for strand passage. DNA Repair (Amst). 2014 Apr;16:23-34. doi: 10.1016/j.dnarep.2014.01.011. Epub 2014 Feb 22. PMID: 24674625. [CrossRef]
- Helgesen E, Sætre F, Skarstad K. Topoisomerase IV tracks behind the replication fork and the SeqA complex during DNA replication in Escherichia coli. Sci Rep. 2021 Jan 12;11(1):474. doi: 10.1038/s41598-020-80043-4. PMID: 33436807; PMCID: PMC7803763. [CrossRef]
- Arrouss I, Decaudin D, Choquet S, Azar N, Parizot C, Zini JM, Nemati F, Rebollo A. Cell penetrating peptides as a therapeutic strategy in chronic lymphocytic leukemia. Protein Pept Lett. 2015;22(6):539-46. doi: 10.2174/0929866522666150216115352. PMID: 25687226. [CrossRef]
|
Disclaimer/Publisher’s Note: The statements, opinions and data contained in all publications are solely those of the individual author(s) and contributor(s) and not of MDPI and/or the editor(s). MDPI and/or the editor(s) disclaim responsibility for any injury to people or property resulting from any ideas, methods, instructions or products referred to in the content. |
© 2023 by the authors. Licensee MDPI, Basel, Switzerland. This article is an open access article distributed under the terms and conditions of the Creative Commons Attribution (CC BY) license (http://creativecommons.org/licenses/by/4.0/).