1. Introduction
Hypericum perforatum L. (St. John’s wort) is the most popular medicinal plant worldwide due to а broad diversity of phenolic compounds with pharmacological properties [
1]. The aerial parts of
H. perforatum wild-growing plants represented the main source of naphthodiathrones, acyl-phloroglucinols, flavonol glycosides, flavan-3-ols, and phenolic acids, while roots synthesized significant amounts of xanthones [
2,
3]. The naphthodianthrone hypericin commonly distributed into dark glands on the leaf and petal margins [
4] has been shown as a powerful photosensitizer used in photodynamic therapy of cancer and virus diseases [
5]. In addition, prenylated phloroglucinols and flavonoids possessed antidepressant, anti-inflammatory, antimicrobial, antioxidant, and wound-healing activities [
1,
6]. The xanthones have been proposed as promising compounds with antibacterial, antifungal, and antidiabetic properties [
7,
8]. Taking into account the continuous demand of the pharmaceutical industry for
H. perforatum raw material, many studies have been focused on the evaluation of various factors that influence the chemical composition of Hyperici herba. In this context, phytochemical variability of field-grown plants has been related to the geographical origin, abiotic and biotic environmental factors, contamination issues, as well the genotype, time of harvest, plant development, and physiological stage [
7,
9,
10]. To overcome these confounding factors, various biotechnological techniques have been applied to obtain
H. perforatum raw material with stable amounts of secondary metabolites [
11,
12].
Plant cell and tissue culture technology represents a promising approach for the sustainable production of bioactive metabolites in controlled and aseptic conditions. The main advantage of
in vitro technology over traditional cultivation of medicinal plants includes fast cell growth, pathogen-free plant material, and reliable secondary metabolite production [
13]. Recently, attractive
in vitro strategies have been established to increase the quantity and quality of
H. perforatum secondary metabolites. The optimization of culture conditions, bioreactor technology, elicitation, and genetic transformation have been extensively studied for the overproduction of hypericins, hyperforins, phenylpropanoids, and xanthones in
H. perforatum in vitro cultures [
11,
14,
15]. Even though elicitation represents the commonly used approach for the up-regulation of secondary metabolites in
H. perforatum in vitro cultures, the commercial relevance is still a challenge due to the influence of culture conditions, cell differentiation, elicitor concentration, and post-elicitation duration [
15]. Since the biological activity of plant extracts is greatly influenced by phenylpropanoid and naphthodianthrone contents, the enhancement of their production has been suggested as an important target for the genetic transformation of
H. perofratum [
16].
In the last two decades,
Agrobacterium-mediated transformation has emerged as a novel approach for the modulation of secondary metabolite production in
Hypericum in vitro cultures [
14]. The
H. perforatum has been shown as a recalcitrant species to
A. tumefaciens-mediated transformation due to the presence of antimicrobial compounds that inhibit bacterial growth [
17]. On the other hand,
A. rhizogenes-mediated transformation of
H. perforatum represents a reliable method to obtain hairy root (HR) cultures characterized by fast growth on an auxin-free medium, as well as genetic and biochemical stability [
18]. The
rol and
aux genes from the T-DNA of
A. rhizogenes root-inducing (Ri) plasmid have been successfully integrated into the genome of
H. perforatum [
19,
20,
21] and other
Hypericum species [
22,
23]. Those studies revealed that phenotypic characteristics, bioactive metabolites production, and antioxidant status of
Hypericum HR clones depend on
rol and
aux gene expression and their copy numbers in the plant genome. Our previous studies revealed that the integration of
A. rhizogenes T-DNA genes into transformed cells suppresses reactive oxygen species (ROS) production in
H. perforatum HR cultures through the activation of antioxidant enzyme machinery and up-regulation of phenylpropanoid metabolism [
24,
25,
26]. In this context,
H. perforatum HR cultures were proposed as a perspective system for enhanced production of root-specific compounds, such as phenolic acids, flavonoids, and xanthones [
21,
24,
26,
27,
28]. However,
Hypericum HR cultures did not show capability for hypericins production, since the accumulation of these bioactive compounds is related to the development of dark glands in plant aerial parts [
4]. Several studies have been directed toward the establishment of
Hypericum HR-regenerated shoots as an efficient experimental system for naphthodianthrone production [
12,
29].
To the best of our knowledge,
H. perforatum HR cultures have a great potential for spontaneous regeneration into transgenic shoots upon exposure to light or photoperiod [
24,
29,
30]. The expression of
rol gene loci from
A. rhizogenes Ri-plasmid into plant genome has been related to the unique morphological characteristics of transgenic plants such as dwarfism and increased branching [
31]. Several studies pointed out that
rol gene copy number is the main determinant for phenotypic alterations in
H. perforatum transgenic plants including the number and density of dark glands as the multicellular reservoirs of hypericins [
32,
33]. The production of hypericins in
Hypericum transgenic shoots has been observed to be comparable [
24,
32] or even higher [
20,
30,
33] than non-transgenic shoots. Thus, the screening of various
H. perforatum transgenic shoot clones could be a promising strategy for the selection of superior lines with enhanced production of bioactive metabolites with antioxidant properties.
In this study, fifteen H. perforatum transgenic shoot clones (TS A-TS O) regenerated from the corresponding HR lines (HR A-HR O) were evaluated for the following topics:
- (1)
biomass production (fresh weight, dry weight, fresh weight/dry weight ratio and dry weight yield);
- (2)
phenotypic characteristics (index of compactness and number of dark glands per leaf);
- (3)
production of total phenylpropanoids (phenolics, flavonoids, flavan-3-ols, condensed taninns, and phenolic acids);
- (4)
identification and quantification of naphthodianthrones (hypericin, pseudohypericin, and protopseudohypericim) by UPLC analysis;
- (5)
phenylalanine ammonia lyase and polyphenol oxidase activities;
- (6)
non-enzymatic antioxidant capacity (cupric reducing antioxidant capacity; ferric ions reducing antioxidant power, ABTS radical scavenging activity, lipid peroxidation inhibition);
- (7)
radical scavenging activity (hydrogen peroxide, superoxide anion and hydroxyl radical);
- (8)
antioxidant enzyme activities (guaiacol peroxidase, ascorbate peroxidase, catalase and superoxide dismutase);
- (9)
oxidative stress marker contents (hydrogen peroxide, superoxide anion and malondialdehyde).
2. Materials and Methods
2.1. Establishment of Hypericum perforatum Transgenic Shoots
The protocol for the establishment of fifteen
H. perforatum TS clones (TS A-TS O) from corresponding HR lines (HR A-HR O) was described in our previous study [
30]. The transgenic nature of HR clones was previously confirmed by PCR amplification of
rolB gene [
21]. The segments from all HR lines along with non-transformed roots (NTR) were inoculated on solid hormone-free MS/B
5 medium and exposed to photoperiod conditions of 16-h light and irradiance at 50 μmol·m
2·s
-1. The NTR and HR A-HR O lines exhibited a potential for spontaneous shoot differentiation into corresponding non-transgenic shoots (NTS) and transgenic shoot clones (TS A-TS O). After the determination of regeneration frequency (RF), NTS and TS clones were multiplied on a solid MS/B
5 medium supplemented with 0.2 mg·L
-1 N
6-benzyladenine (BA).
2.2. Growth and Phenotypic Characteristics
The NTS and TS clones were used for the determination of fresh weight (FW), dry weight (DW), FW/DW ratio, and dry weight yield (DWY). The morphology of shoot cultures was evaluated by measurement of shoot length (SL) and number of leaves per shoot (NLS). These parameters were used to calculate the index of compactness (IC=SL/NLS) of shoot cultures. Fully developed apical leaf couples were used for the determination of the number of dark glands per leaf (NDG).
2.3. Phenylpropanoid Production
The
H. perforatum control (NTS) and transgenic shoot lines (TS A-TS O) were harvested, frozen in liquid nitrogen or lyophilized, and then stored at -80°C, until phytochemical analyses. Briefly, phenolic compounds were extracted from powdered dry plant material with 80% (v/v) CH
3OH in an ultrasonic bath for 30 min at 4°C [
34,
35]. Thereafter, methanolic extracts were centrifuged at 12000 rpm for 15 min and the supernatants were used for the determination of total contents of phenolics (TP), flavonoids (TF), flavan-3-ols (TFA), condensed tannins (TCT), and phenolic acids (TPA). The protocols for quantification of TP, TF, TFA, TCT, and TPA in shoot extracts were described in our recent studies [
25,
26]. Spectrophotometric analyses were performed on SpectraMax 190 Microplate Reader (Molecular Devices Corp., Sunnyvale, CA) supported with SoftMax Pro (v. 5.4.1) software.
2.4. UPLC-TUV Analysis of Naphthodianthrones
Extract preparation. The extracts were prepared by homogenization of 0.05 g lyophilized shoot cultures with 1 mL 80% (v/v) CH3OH in an ultrasonic bath for 30 min. The homogenates were vortexed and centrifuged at 12000 rpm for 15 min at 4°C. The supernatants were filtered through 0.2 µm filters (Ministart RC 25, Sartorius Stedim Biotech, Germany) prior to the chromatographic analysis. The light was excluded as much as possible during all procedures for extract preparation.
UPLC-TUV system. The identification and quantification of naphthodianthrones in shoot extracts were performed on ACQUITY ultra-performance liquid chromatography (UPLC) system with dual-wavelength tunable UV/Vis (TUV) detector (H-class, Waters, Milford, MA, USA). The instrument was equipped with a quaternary solvent manager (QSM), sample manager-flow through the needle (SM-FTN), degassing system, and CH-A column heater controlled by ACQUITY UPLC Console and MassLynx software (Waters). Chromatographic separation of naphthodianthrones was performed using ACQUITY UPLC HSS T3 column (100 × 2.1 mm, 1.8 μm; Waters) thermostated at 38°C. The mobile phase consisted of two solvents A: 0.1% formic acid (v/v) and B: acetonitrile. The gradient program was as follows: from 10% to 50% B (0.0–1.0 min), from 50% to 100% B (1.0–14 min), 100% B (14–19 min), and from 100% to 10% B (19–25 min). The flow rate was set at 0.571 mL·min−1 and the injection volume was 10 μL. Total run time was 25 min. Retention time (tR) for protopseudohypericin (PPHYP), pseudohypericin (PHYP), and hypericin (HYP) were 3.9, 4.9, and 12.6 min, respectively.
The peak assignment of naphthodianthrones was based on the comparison of their retention time to those of reference standards. The reference standards of hypericin (HYP) and pseudohypericin (PHYP) were HPLC grade with >99% and >98% purity, respectively (PLANTA Naturstoffe Vertriebs GmbH, Vienna, Austria). The stock solutions of reference standards (1 mg·mL-1) were prepared in 80% (v/v) CH3OH and were stored at 4°C. The external standard method was used for quantification of all identified naphthodianthrones in shoot samples at 590 nm according to their peak area.
The identification of naphthodianthrones in shoot extracts was additionally confirmed using the HPLC system connected to the Agilent G2445A ion-trap mass spectrometer equipped with electrospray ionization (ESI) system and controlled by LCMSD software (Agilent, v.6.1.) as previously reported [
24,
30,
36]. The UV/Vis, MS, and MS
2 spectra of the component peaks are presented in
Table 1.
Method validation. The UPLC-TUV method was validated for linearity, sensitivity, accuracy and precision. To determine the linearity and range of the analytical method, the standard stock solutions (1 mg·mL
-1) for HYP and PHYP were diluted in concentration ranges of 0.02-0.1 μg·mL
-1 and 0.1-3 μg·mL
-1, respectively. Calibration curves of HYP and PHYP revealed a good linearity, as shown by the correlation coefficients above 0.99 in tested ranges (
Table 2). The sensitivity of the method was evaluated by determination of the limit of detection (LOD) and limit of quantification (LOQ). The LOD and LOQ values (
Table 2) were calculated using the following formulas: LOD = 3.3 × σ/S and LOQ = 10 × σ/S, where σ is the standard deviation of the
y-intercept and S is the slope of the calibration curve in the low concentration level (0.001-0.01 μg·mL
-1 for HYP and 0.01-0.2 μg·mL
-1 for PHYP). The accuracy of the method was assessed through a recovery test. The contents of HYP and PHYP in shoot samples were 0.025 and 1.918 μg·mL
-1, respectively. Then, three different concentration levels (high, medium, and low) of a standard mixture were added to this sample (
Table 2).
The spiked samples were then analyzed with the previously established method. The recovery was calculated according to the following formula: Recovery = (found concentration-original concentration)/spiked concentration × 100%. The recoveries for HYP and PHYP are presented in
Table 2. To determine the repeatability of the method, six replicates of mixed standard solutions containing PHYP and HYP at three different concentrations within the concentration range were injected and analyzed. The relative standard deviation (RSD) of the components peak area was < 1.5% (
Table 2). These data demonstrated that the established UPLC method had satisfactory precision, accuracy, and repeatability for simultaneous analysis of HYP and PHYP in
H. perforatum shoot culture extracts.
2.5. Phenylalanine Ammonia Lyase (PAL) and Polyphenol Oxidase (PPO) Activities
The extracts for the determination of phenylalanine ammonia lyase (PAL) and polyphenol oxidase (PPO) activities were prepared by homogenization of frozen shoot tissue (about 0.3 g) in the appropriate buffer solution. For the PAL assay was used 1 mL 100 mМ sodium borate (Na
2B
4O
7, pH 8.8), while for the PPO assay was used 50 mM K
2HPO
4/KH
2PO
4 (pH 7.0) buffer [
26]. The enzyme extracts were also used for the determination of protein content by Bradford assay using bovine serum albumin as a standard [
37].
The PAL assay was performed in a reaction mixture consisting of shoot extract and 20 mM L-phenylalanine [
34,
35]. The molar extinction coefficient of
trans-cinnamic acid (ε
290=9630 L·mol
-1·cm
-1) was used to express PAL activity in pkat·mg
-1 proteins.
The PPO assay was conducted by mixing the shoot extract and 40 mM pyrocatechol [
26]. The molar extinction coefficient of
ortho-benzoquinone (ε
390=1417 L·mol
-1·cm
-1) was used to express PPO activity in nkat·mg
-1 proteins.
2.6. Antioxidant Activities
The antioxidant activity was determined in methanolic shoot extracts by the following methods: cupric ion-reducing antioxidant capacity (CUPRAC), ferric ion-reducing antioxidant power (FRAP), ABTS radical scavenging activity and lipid peroxidation inhibition (LPI). The CUPRAC and LPI assays were performed according to the protocols described in our previous studies [
25,
26].
The FRAP assay was determined in a reaction mixture including shoot extract and FRAP reagent [
38]. The FRAP reagent consisted of 300 mM CH
3COONa buffer (pH 3.6), 10 mM TPTZ (2,4,6-tri(2-pyridyl)-s-triazine) in 40 mM HCl and 20 mM FeCl
3 (10:1:1, v/v/v). After incubation of the reaction mixture for 30 min at 37°C under dark, the sample absorbance was measured at 593 nm. The FRAP values were calculated with FeSO
4·7H
2O as a standard (0-10 mМ). Results were expressed as micromoles of Fe
2+ equivalents per gram of dry weight (μM Fe
2+·g
-1 DW).
The ABTS radical scavenging activity was determined by the method of Re et al. [
39]. The ABTS radical cation (ABTS
•+) was generated by a reaction of 7 mM ABTS [2.2’-azinobis(3-ethylbenzothiazoline-6-sulphonic acid) diammonium salt] with 2.45 mM K
2S
2O
8 (1:1, v/v). The mixture was incubated in the dark (12–16 h) at room temperature and the generated blue-green ABTS
•+ solution was diluted with CH
3OH to obtain an absorbance of 0.70 (±0.05) at 734 nm. All shoot extracts were diluted to give a 20-80% absorbance decrease of ABTS
•+ solution. The reaction was initiated by the addition of ABTS
•+ solution to shoot extract and thoroughly mixed. The control samples contained CH
3OH instead of plant extract. The samples were allowed to stand for 6 min and the absorbance was recorded at 734 nm. The ABTS radical scavenging activity was calculated with trolox as a standard (0-1000 μМ). Results were expressed as micromoles of Trolox equivalents (T) per gram of dry weight (μM T·g
-1 DM).
2.7. Radical Scavenging Activities
The scavenging activity (SA) of shoot extracts was determined for the hydrogen peroxide (H2O2), superoxide radical (O2•-), and hydroxyl radical (OH•).
The H
2O
2 SA and O
2•- SA were performed in shoot methanolic extracts as previously described in our studies [
25,
26], while OH
• SA was evaluated in water extracts with the method described by [
40].
The protocol for OH• SA was performed in the reaction mixture consisted of shoot extract, 28 mM 2-deoxy-2-D-ribose (in 20 mM KH2PO4/KOH buffer, pH 7.4), 0.1 mM EDTA, 0.1 mM FeCl3, 1 mM ascorbic acid (AA) and 1 mM H2O2. After incubation at 37°С for 1 hour, 1% trichloroacetic acid (TCA) and 2.8% thiobarbituric acid (TBA) were added and the mixture was incubated at 95°С for 30 min. The absorbance of pink colored complex was measured at 532 nm. The OH• SA of shoot extracts was calculated with mannitol as a standard (0-10 mМ). Results were expressed as millimoles of mannitol equivalents (M) per gram of dry weight (mM M·g-1 DW).
2.8. Antioxidant Enzymes
Antioxidant enzymes such as guaiacol peroxidase (PX), ascorbate peroxidase (APX), catalase (CAT), and superoxide dismutase (SOD) were determined in shoot extracts. The procedure for enzyme extraction was performed by the method described in our previous study [
26].
The PX activity was measured in a reaction mixture consisting of enzyme extract, 2% guaiacol, and 0.3% H
2O
2 [
41]. The rate of absorbance increase due to tetraguaiacol formation was monitored for a period of 5 min at 470 nm. The molar extinction coefficient of tetraguaiacol (ε
470=26.6 L·mol
-1·cm
-1) was used to express PX activity in nkat·mg
-1 proteins.
The APX activity was determined by mixing enzyme extract with 0.5 mM AA and 1 mM H
2O
2 [
42]. The rate of absorbance decrease due to AA oxidation was monitored for a period of 10 min at 290 nm. The molar extinction coefficient of AA (ε
290=2.8 L·mol
-1·cm
-1) was used to express APX activity in pkat·mg
-1 proteins.
The CAT activity was performed in a reaction mixture consisting of enzyme extract, 0.1% H
2O
2, and 50 mM potassium phosphate buffer [
43]. The rate of absorbance decrease due to H
2O
2 consumption was monitored for a period of 1 min at 240 nm. The molar extinction coefficient of H
2O
2 (ε
240=43.6 L·mol
-1·cm
-1) was used to express CAT activity in nkat·mg
-1 proteins.
The SOD activity was measured through the determination of the sample’s ability to inhibit photochemical reduction of NBT [
44]. The reaction mixture consisting of enzyme extract, 130 mM methionine, 750 μM NBT, and 20 μM riboflavin was illuminated for 15 min. The absorbance of the illuminated reaction mixture was recorded at 560 nm and compared with that of the non-illuminated reaction mixture that served as a control. One unit (U) of SOD activity was defined as the amount of enzyme required to cause 50% inhibition of NBT photoreduction rate. The SOD activity was expressed as U·mg
-1 protein.
2.9. Oxidative Stress Markers
The shoot extracts for quantification of oxidative stress markers H2O2 and malondialdehyde (MDA) were prepared from fresh tissue using 5% ТСА, while O2•- production rate was assessed in the extracts for determination of antioxidant enzymes.
The endogenous H
2O
2 level was determined in the reaction mixture consisting of shoot extract, 10 mM K
2HPO
4/KH
2PO
4 buffer (pH 7.0), and 1 М KI [
45]. After incubation at room temperature for 1 hour, the sample absorbance was measured at 390 nm. The H
2O
2 content was calculated using H
2O
2 as a standard (0-1000 μМ). The results were expressed as micromoles of H
2O
2 per gram of fresh weight (μM H
2O
2·g
-1 FW).
The O
2•- production rate was measured in a mixture containing shoot extract, 50 mM potassium phosphate buffer (pH 7.0), and 10 mM hydroxylamine hydrochloride [
46]. Thereafter, an aliquot of Griess reagent was added to the reaction mixture and the absorbance was measured at 530 nm. The O
2•- production rate was calculated with NaNO
2 as a standard (0-1000 μМ). The results were expressed as nanomoles of generated O
2•- per min and gram of fresh weight (nM O
2•- ·min
-1·g
-1 FW).
The MDA content was determined by mixing shoot extracts with 0.5% TBA in 20% TCA and further heating of reaction mixture at 95°C for 30 min [
47]. After cooling to room temperature, the sample absorbance was monitored at 532 nm and at 600 nm to subtract the non-specific absorbance. The MDA content was calculated using the molar extinction coefficient of MDA (155 mM
-1·cm
-1) and the results were expressed as nanomoles MDA per gram of fresh weight (nМ MDA·g
-1 FW).
2.10. Statistical Analysis
The experiments with transgenic shoot clones were independently repeated two times under the same conditions and all analyses were performed in triplicate. All data were presented as mean values with standard deviation (±SD). The data were analyzed by one-way ANOVA (version 5.0; StatSoft Inc., Tulsa, OK, USA) to detect significant differences between shoot samples. A post hoc separation of means between different shoot clones was performed by the Duncan’s test (p < 0.05). The data for growth and morphological characteristics, phenylpropanoid and naphthodianthrone contents, as well for the antioxidant status were subjected to principal component analysis (PCA) and hierarchical agglomerative clustering (HAC) using the statistical software XLSTAT 2014.5.03 (Addinsoft, NY, USA). Pearson’s correlation coefficients between the analyzed parameters were obtained by R software v. 4.2.1 (R Core Team, Vienna, Austria).
3. Results
3.1. Establishment of Hypericum perforatum Transgenic Shoots
Transgenic shoot clones (TS A-TS O) of
H. perforatum were regenerated from corresponding hairy root clones (HR A-HR O) grown on hormone-free MS/B
5 medium under photoperiod (
Figure 1A). Non-transgenic shoots (NTS) that served as a control were regenerated from non-transgenic root cultures (NTR). The regenerated shoots were isolated from root tissues and then cultivated on a medium supplemented with 0.2 mg·L
-1 BA for incessant growth. After 1 month of cultivation, shoot clones were successfully multiplied (
Figure 1B) and were evaluated for differences in morphological and growth characteristics, production of phenylpropanoids and naphthodianthrones, as well antioxidant status.
3.2. Morphological and Growth Characteristics of Transgenic Shoots
The cultivation of HR and NTR explants on hormone-free MS/B
5 solid medium resulted in spontaneous regeneration into corresponding TS clones and NTS. The RF of HR and NTR explants was evaluated after 1 month of cultivation (
Table 3). The HR clones showed significant differences in RF from 12.5% to 82%. A maximum RF was observed for HR G and HR L clones, which was about 4-fold higher in comparison to NTR. In contrast, HR C and HR J clones showed significantly lower RF (1.6-fold) compared to NTR. Multiplied TS clones and NTS cultures of
H. perforatum were used for the evaluation of biomass accumulation FW, DW, FW/DW ratio, and DWY, as well as morphological characteristics including the IC and NDG (
Table 2).
The FW production among TS clones varied from 2.16 to 18.68 g. The FW accumulation in TS D and TS K was markedly higher (about 3.5-fold) than that found in NTS. Results also showed variability in DW production among TS clones from 0.21 to 0.98 g. The highest DW production was noticed in TS K, which was about 2.9-fold of that observed in NTS. The FW/DW ratio of TS clones was ranged from 8.83 to 25.06. The TS C and TS D clones showed a significantly higher FW/DW ratio (about 1.6-fold) than that observed in NTS. With respect to DWY in TS clones, the values varied from 3.99% to 11.33%. The TS A and TS N clones displayed about 1.7-fold higher DWY than that noticed in NTS. The IC in TS clones varied from 2.40 to 5.27, while NTS had a value of 3.73. Only three clones (TS G, TS I, and TS M) showed significantly higher IC values (up to 1.4-fold) compared to NTS. The NDG in TS clones was ranged from 6.00 to 15.67, while NTS showed a value of 9.00. Three clones denoted as TS B, TS G, and TS K exhibited the highest NDG value (from 1.5- to 1.7-fold) than that found in control shoots.
3.3. Phenylpropanoid Production in Transgenic Shoots
The results for phenylpropanoid contents, as well as for phenylalanine ammonia lyase (PAL) and polyphenol oxidase (PPO) activities in
H. perforatum shoot cultures are presented in
Table 4. The contents of phenolics (TP) in TS clones ranged from 23.26 to 62.03 mg GA·g
-1 DW. Five clones denoted as TS C-TS F and TS J showed significantly higher TP amounts (up to 1.4-fold) compared to NTS. Total flavonoid (TF) contents in TS clones showed values from 7.36 to 27.82 mg C·g
-1 DW. Three clones TS C, TS D, and TS F exhibited the highest TF production (about 1.4-fold) than that found in NTS. The amounts of flavan-3-ols (TFA) in TS clones varied from 1.85 to 10.44 mg C·g
-1 DW. The TS F clone showed 2-fold elevated TFA production compared to NTS. The TS clones displayed a variation in condensed tannins (TCT) contents (1.37-4.2 mg CG·g
-1 DW). The highest TCT production (1.3-fold) was noticed for the TS F clone in comparison to NTS. Total phenolic acids (TPA) content in TS clones varied from 1.29 to 4.35 mg P·g
-1 DW. Two clones TS E and TS F showed slightly higher TPA contents than that found in NTS.
The PAL activity in TS clones varied from 0.1 to 2.8 pkat·mg-1 P. Three clones denoted as TS A, TS F, and TS N showed significantly higher enzyme activity (from 1.3- to 3.9-fold) compared to NTS. Present results demonstrated that all tested TS clones had markedly enhanced PPO activity (11.49-51.39 nkat·mg-1 P) compared to NTS (5.6 nkat·mg-1 P). A maximum increase in PPO activity was noticed in TS A and TS C clones which was about 9.2-fold higher than that found in NTS cultures.
3.4. Naphthodianthrone Production in Transgenic Shoots
Among the group of naphthodianthrones, hypericin (HYP), pseudohypericin (PHYP), and protopseudohypericin (PPHYP) were identified and quantified in all tested TS clones, as well in NTS (
Table 5). Particularly, PHYP was found as a pre-eminent compound from the class of naphthodianthrones in shoot extracts (
Figure 2).
The HYP contents in TS clones ranged from 11.11 to 69.41 µg·g-1 DW. From the selected clones, only TS B showed markedly higher HYP amounts (2.4-fold) compared to NTS. The amounts of PHYP in TS clones varied from 537.09 to 1915.34 µg·g-1 DW. The most of TS clones exhibited significantly higher or comparable PHYP contents than in NTS. A maximum PHYP production was observed in the TS B clone that was almost 3-fold enhanced than NTS. The PPHYP contents in TS clones varied from 142.61 to 578.80 µg·g-1 DW. It is interesting to note that all tested TS clones displayed significantly increased PPHYP amounts compared to NTS cultures. The highest PPHYP production (6.3-fold) was noticed for the TS H clone than that observed in NTS cultures.
3.5. Antioxidant and Radical Scavenging Activities in Transgenic Shoots
The
H. perforatum shoot cultures were evaluated for antioxidant capacity (CUPRAC, FRAP, ABTS, and LPI) and radical scavenging activity (H
2O
2 SA, O
2•- SA, and OH
• SA), (
Table 6).
The CUPRAC values in TS clones varied from 79.56 to 250.81 μМ Т·g-1 DW. The highest value for CUPRAC (1.4-fold) was found in TS F and TS E clones compared to NTS. The FRAP values in TS clones ranged from 230.88 to 649.91 μМ Fe2+·g-1 DW. A maximum FRAP was noticed in the TS F clone (1.5-fold) compared to NTS cultures. The ABTS values in TS cultures varied from 48.66 to 168.75 μМ Т·g-1 DW and the TS F clone showed 1.4-fold higher activity compared to NTS. The LPI in TS clones varied from 51.50 to 91.33% and only the TS K clone exhibited a slightly higher value compared to NTS cultures.
The H2O2 SA in all tested TS clones (21.92-33.15%) was significantly higher compared to NTS (18.47%). A maximum H2O2 SA was noticed in the TS K clone (1.8-fold) than that found in NTS. The O2•- SA in TS clones varied from 24.20 to 57.94% and the highest value was noticed in the TS N clone (1.7-fold) in comparison to NTS (34.68%). The TS clones did not show a great variation in OH• SA (1.14-1.85 mM M·g-1 DW), and only the TS F clone displayed a slightly higher scavenging value compared to NTS cultures.
3.6. Antioxidant Enzymes and Oxidative Stress Markers in Transgenic Shoots
The enzymatic antioxidant system in
H. perforatum shoot extracts was evaluated through the activity of guaiacol peroxidase (PX), ascorbate peroxidase (APX), catalase (CAT) and superoxide dismutase (SOD), while oxidative stress markers included quantification of H
2O
2, O
2•- and malondialdehyde (MDA), (
Table 7).
The PX activity in all tested TS clones (0.04-0.29 nkat·mg-1 P) was significantly higher compared to NTS (0.03 nkat·mg-1 P). The TS B clone showed markedly higher PX activity (9.7-fold) compared to NTS. The APX activity in TS clones varied from 13.06 to 124.69 pkat·mg-1 P and only TS C and TS A showed significantly higher enzyme activity (1.9- and 1.6-fold, respectively) compared to NTS. The CAT activity in TS clones ranged from 0.11 to 1.17 nkat·mg-1 P and TS G and TS C showed significantly elevated enzyme activity (1.5- and 1.3-fold, respectively) in comparison to NTS. The SOD activity in TS clones varied from 0.47 to 7.51 U·mg-1 P and TS C and TS A exhibited significantly enhanced SOD activity (3.7- and 1.2-fold, respectively) than that in NTS.
The H2O2 contents in all TS clones (0.38-1.18 μM·g-1 FW) were significantly decreased compared to NTS (1.34 μM·g-1 FW). The TS A clone showed markedly lower H2O2 contents (3.5-fold) compared to NTS cultures. The O2•- production rate in TS clones varied from 0.24 to 1.22 nM·min-1·g-1 FW and five clones (TS I, TS J, TS L, TS M, and TS O) exhibited significantly higher values (up to 1.9-fold) compared to NTS. Other TS clones demonstrated comparable or lower O2•- production rate than NTS. The MDA contents in TS clones (0.41 to 1.75 nM·g-1 FW) were similar or lower compared to NTS (1.42 nM·g-1 FW).
3.7. Principal Component Analysis and Hierarchical Agglomerative Clustering
The principal component analysis (PCA) and hierarchical agglomerative clustering (HAC) were performed as statistical tools to determine the variance of analyzed parameters within fifteen
H. perforatum TS clones and NTS, as well as to cluster the samples (
Figure 3).
The PCA data showed that PC1 and PC2 explained 49.74% of the total variation. The loadings plot of PCA (
Figure 3A) indicated that the PC1 exhibited a variance of 32.67% that was positively related to phenylpropanoid production (TP, TF, TFA, TCT, TPA), antioxidant activities (CUPRAC, FRAP, ABTS) and OH
• SA. The PC2 showed a variance of 17.07% and it was positively related to the growth and morphological characteristics (FW, DW, FW/DW, NDG), but a negative relationship was observed for DWY.
Present results for the analyzed parameters showed that control and transgenic shoots are mainly separated on PC1 (
Figure 3B) and grouped into 3 clusters (
Figure 3C). Cluster I was represented with five clones (TS C-TS F, TS J) along with NTS exhibiting positive scores on PC1. Those TS clones were described with intensive phenylpropanoid metabolism that resulted in the accumulation of phenolic compounds with antioxidant and OH
• scavenging capacity. Cluster II included six clones (TS A, TS B, TS G, TS H, TS K, TS L) distributed among the origin of PC1 and they were characterized by moderate production of phenylpropanoids and antioxidant activities. Cluster III was represented by four clones (TS I, TS M-TS O) displaying the strongest negative scores on PC1 and they showed weak phenylpropanoid metabolism and low non-enzymatic antioxidant status.
The Pearson’s correlation matrix (
Figure 4) demonstrated that phenylpropanoids (TP, TPA, TF, TCT, and TFA) were in significant positive correlation with growth characteristics (FW and FW/DW), antioxidant/radical scavenging activity (CUPRAC, FRAP, ABTS, and OH
• SA) and antioxidant enzymes (PX, APX, CAT, and SOD). The activities of antioxidant enzymes including PPO showed a significant negative correlation with oxidative stress markers (H
2O
2 and O
2). The H
2O
2 and MDA levels showed a significant negative correlation with naphthodianthrone production (HYP, PHYP, and PPHYP). The O
2•- production rate was in significant negative correlation with phenylpropanoid production and antioxidant activities. The contents of identified naphthodianthrones were in significant positive correlation with growth and phenotypic characteristics (RF, FW, DW, FW/DW, and NDG), as well as with antioxidant enzymes (GPX and CAT).
Author Contributions
Conceptualization, methodology, formal analysis, investigation, resources, data curation, visualization, writing—review and editing, O.T. and S.G.S.; validation, software, formal analysis, M.T. and I.T.; methodology, formal analysis, investigation, validation, J.P.S.; writing—original draft preparation, O.T.; supervision, S.G.S. All authors have read and agreed to the published version of the manuscript.
Figure 1.
Hypericum perforatum transgenic shoots (TS) regenerated from hairy roots (HR) on a hormone-free medium (A). Multiplication of TS on MS/B5 medium supplemented with 0.2 mg·L-1 N6-benzyladenine (B).
Figure 1.
Hypericum perforatum transgenic shoots (TS) regenerated from hairy roots (HR) on a hormone-free medium (A). Multiplication of TS on MS/B5 medium supplemented with 0.2 mg·L-1 N6-benzyladenine (B).
Figure 2.
Chromatograms of Hypericum perforatum control (A) and transgenic shoots (B) monitored at 590 nm for detection of naphthodianthrones. HYP: hypericin; PHYP: pseudohypericin; PPHYP: protopseudohypericin.
Figure 2.
Chromatograms of Hypericum perforatum control (A) and transgenic shoots (B) monitored at 590 nm for detection of naphthodianthrones. HYP: hypericin; PHYP: pseudohypericin; PPHYP: protopseudohypericin.
Figure 3.
Loadings plot (A) and scores plot (B) of principal component analysis and hierarchical agglomerative clustering (C) for growth and morphological characteristics, phenylpropanoid and naphthodianthrone production, as well antioxidant status of Hypericum perforatum transgenic shoots. Note: PC: principal components; NTS: control shoots; TS A-TS O: transgenic shoot clones; TP: total phenolics; TF: total flavonoids; TFA: total flavan-3-ols; TCT: total condensed tannins; TPA: total phenolic acids; CUPRAC: cupric reducing antioxidant capacity; FRAP: ferric ion reducing antioxidant power, ABTS: ABTS radical scavenging activity; LPI: lipid peroxidation inhibition; H2O2 SA: hydrogen peroxide scavenging activity, O2•− SA: superoxide anion scavenging activity, OH• SA: hydroxyl radical scavenging activity; PAL: phenylalanine ammonia lyase; PPO: polyphenol oxidase; PX: guaiacol peroxidase; APX: ascorbate peroxidase; CAT: catalase; SOD: superoxide dismutase; H2O2: hydrogen peroxide; O2•-: superoxide anion; MDA: malondialdehyde; RF: regeneration frequency; FW: fresh weight, DW: dry weight, FW/DW: fresh weight/dry weight ratio; DWY: dry weight yield; IC: index of compactness; NDG: number of dark glands, HYP: hypericin; PHYP: pseudohypericin; PPHYP: protopseudohypericin.
Figure 3.
Loadings plot (A) and scores plot (B) of principal component analysis and hierarchical agglomerative clustering (C) for growth and morphological characteristics, phenylpropanoid and naphthodianthrone production, as well antioxidant status of Hypericum perforatum transgenic shoots. Note: PC: principal components; NTS: control shoots; TS A-TS O: transgenic shoot clones; TP: total phenolics; TF: total flavonoids; TFA: total flavan-3-ols; TCT: total condensed tannins; TPA: total phenolic acids; CUPRAC: cupric reducing antioxidant capacity; FRAP: ferric ion reducing antioxidant power, ABTS: ABTS radical scavenging activity; LPI: lipid peroxidation inhibition; H2O2 SA: hydrogen peroxide scavenging activity, O2•− SA: superoxide anion scavenging activity, OH• SA: hydroxyl radical scavenging activity; PAL: phenylalanine ammonia lyase; PPO: polyphenol oxidase; PX: guaiacol peroxidase; APX: ascorbate peroxidase; CAT: catalase; SOD: superoxide dismutase; H2O2: hydrogen peroxide; O2•-: superoxide anion; MDA: malondialdehyde; RF: regeneration frequency; FW: fresh weight, DW: dry weight, FW/DW: fresh weight/dry weight ratio; DWY: dry weight yield; IC: index of compactness; NDG: number of dark glands, HYP: hypericin; PHYP: pseudohypericin; PPHYP: protopseudohypericin.
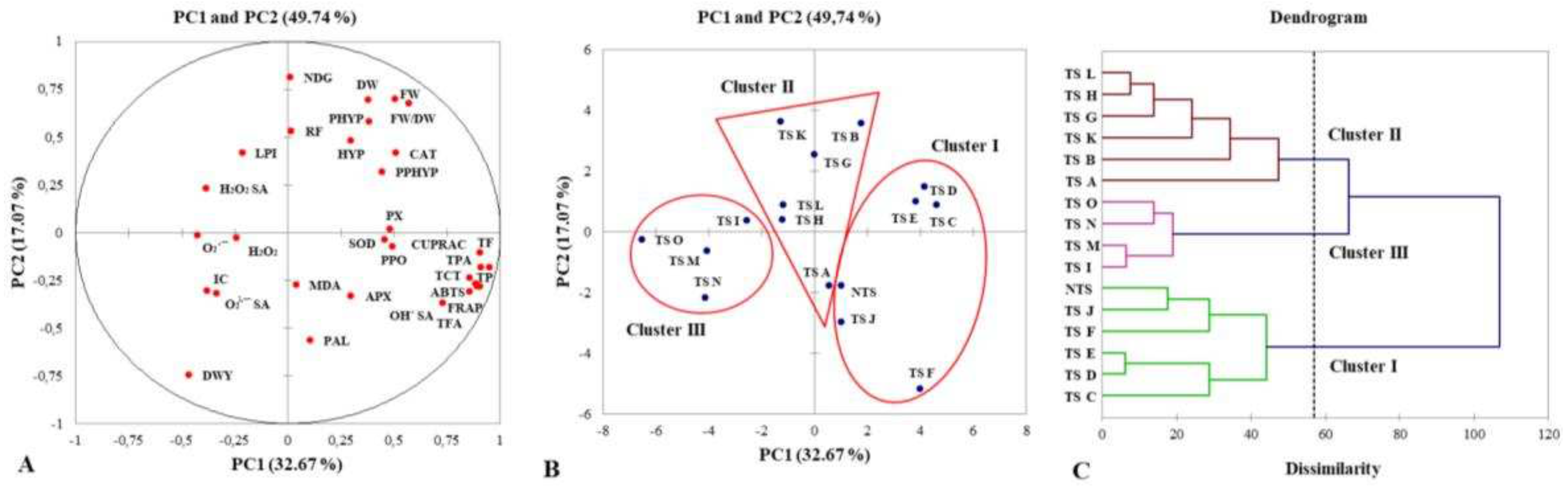
Figure 4.
Correlation matrix between growth and morphological characteristics, phenylpropanoid and naphthodianthrone production, as well antioxidant status of Hypericum perforatum transgenic shoots. Blue colors indicated positive correlation, while red colors showed negative correlation. Note: TP: total phenolics; TF: total flavonoids; TFA: total flavan-3-ols; TCT: total condensed tannins; TPA: total phenolic acids; CUPRAC: cupric reducing antioxidant capacity; FRAP: ferric ion reducing antioxidant power, ABTS: ABTS radical scavenging activity; LPI: lipid peroxidation inhibition; H2O2 SA: hydrogen peroxide scavenging activity, O2•− SA: superoxide anion scavenging activity, OH• SA: hydroxyl radical scavenging activity; PAL: phenylalanine ammonia lyase; PPO: polyphenol oxidase; PX: guaiacol peroxidase; APX: ascorbate peroxidase; CAT: catalase; SOD: superoxide dismutase; H2O2: hydrogen peroxide; O2•-: superoxide anion; MDA: malondialdehyde; RF: regeneration frequency; FW: fresh weight, DW: dry weight, FW/DW: fresh weight/dry weight ratio; DWY: dry weight yield; IC: index of compactness; NDG: number of dark glands, HYP: hypericin; PHYP: pseudohypericin; PPHYP: protopseudohypericin.
Figure 4.
Correlation matrix between growth and morphological characteristics, phenylpropanoid and naphthodianthrone production, as well antioxidant status of Hypericum perforatum transgenic shoots. Blue colors indicated positive correlation, while red colors showed negative correlation. Note: TP: total phenolics; TF: total flavonoids; TFA: total flavan-3-ols; TCT: total condensed tannins; TPA: total phenolic acids; CUPRAC: cupric reducing antioxidant capacity; FRAP: ferric ion reducing antioxidant power, ABTS: ABTS radical scavenging activity; LPI: lipid peroxidation inhibition; H2O2 SA: hydrogen peroxide scavenging activity, O2•− SA: superoxide anion scavenging activity, OH• SA: hydroxyl radical scavenging activity; PAL: phenylalanine ammonia lyase; PPO: polyphenol oxidase; PX: guaiacol peroxidase; APX: ascorbate peroxidase; CAT: catalase; SOD: superoxide dismutase; H2O2: hydrogen peroxide; O2•-: superoxide anion; MDA: malondialdehyde; RF: regeneration frequency; FW: fresh weight, DW: dry weight, FW/DW: fresh weight/dry weight ratio; DWY: dry weight yield; IC: index of compactness; NDG: number of dark glands, HYP: hypericin; PHYP: pseudohypericin; PPHYP: protopseudohypericin.
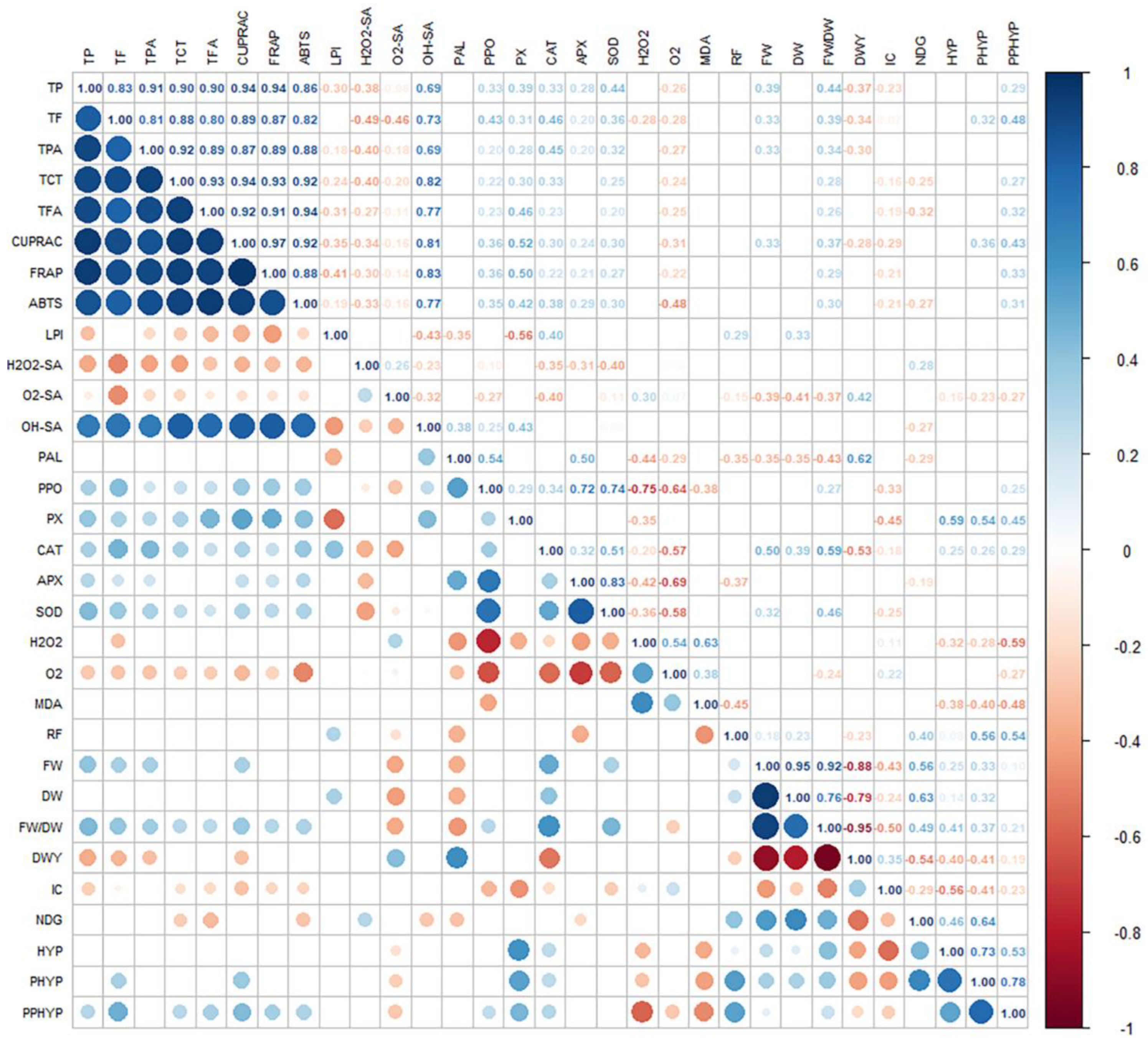
Table 1.
The UV and mass spectral data of naphthodianthrones in Hypericum perforatum shoot extracts.
Table 1.
The UV and mass spectral data of naphthodianthrones in Hypericum perforatum shoot extracts.
Compounds |
UV (nm) |
[M-H]‒ (m/z) |
-MS2 [M-H] ‒ (m/z) |
PPHYP |
285, 375, 550 |
521 |
423 |
PHYP |
288, 325, 465, 580 |
519 |
487, 421 |
HYP |
288, 325, 465, 580 |
503 |
405 |
Table 2.
Linear regression equation, correlation coefficient, sensitivity, accuracy, and repeatability of hypericin (HYP) and pseudohypericin (PHYP) by UPLC analysis.
Table 2.
Linear regression equation, correlation coefficient, sensitivity, accuracy, and repeatability of hypericin (HYP) and pseudohypericin (PHYP) by UPLC analysis.
Compound |
Test range (μg·mL-1) |
Linear regression equation |
R2
|
LOD (μg·mL-1) |
LOQ (μg·mL-1) |
Spiked conc. (μg·mL-1) |
Recovery (%) |
Average Recovery (%) |
RSD (%) |
PHYP |
0.1-3 |
y=334.76x-26.28 |
0.997 |
0.0086 |
0.0260 |
1.534 |
114.98 |
103.15 |
1.27 |
1.918 |
97.68 |
2.302 |
96.79 |
HYP |
0.02-0.1 |
y=788x-7.96 |
0.998 |
0.0005 |
0.0016 |
0.020 |
90 |
97.77 |
1.40 |
0.025 |
100 |
0.030 |
103.30 |
Table 3.
Morphological and growth characteristics of Hypericum perforatum transgenic shoots.
Table 3.
Morphological and growth characteristics of Hypericum perforatum transgenic shoots.
Root cultures |
RF (%) |
Shoot cultures |
FW (g) |
DW (g) |
FW/DW |
DWY (%) |
IC (NLS/SL) |
NDG |
NTR |
20.00±1.50 b-e
|
NTS |
5.28±0.59 bc
|
0.34±0.02 d
|
15.48±1.24 e
|
6.46±0.32 de
|
3.73±0.33 cdg
|
9.00±1.83 bc
|
HR A |
26.60±0.80 g |
TS A |
2.41±0.33 a
|
0.27±0.03 bc
|
8.83±0.38 a
|
11.33±1.00 n
|
3.43±0.39 bd
|
9.75±0.50 be
|
HR B |
20.00±2.00 b-df |
TS B |
13.70±1.23 e |
0.63±0.01 fg
|
21.81±1.57 gh
|
4.58±0.20 ac
|
2.40±0.11 a
|
15.67±3.21 lm
|
HR C |
12.50±1.20 a
|
TS C |
17.47±2.51 f
|
0.70±0.04 i
|
25.06±1.45 h
|
3.99±0.11 a
|
3.31±0.14 bc
|
10.75±1.50 bh
|
HR D |
45.00±2.10 hi
|
TS D |
18.60±3.45 f
|
0.77±0.05 j
|
24.10±1.31 h
|
4.15±0.30 ab
|
3.11±0.16 be
|
10.67±1.15 bg |
HR E |
50.00±3.80 i
|
TS E |
14.17±1.66 e
|
0.76±0.02 j
|
18.58±1.29 f
|
5.38±0.19 cd
|
3.68±0.17 cd
|
12.50±2.08 c-k
|
HR F |
15.00±0.70 ac
|
TS F |
2.16±0.22 a
|
0.21±0.01 a
|
10.31±1.04 ab
|
9.69±0.09 lm
|
4.23±0.41 f-k
|
6.00±1.00 a
|
HR G |
78.00±5.60 k
|
TS G |
8.57±0.14 d
|
0.59±0.03 f
|
14.61±1.23 de
|
6.85±0.50 eh
|
4.72±0.03 kl
|
14.00±1.63 i-km
|
HR H |
44.00±2.40 h
|
TS H |
4.05±0.28 ab
|
0.32±0.01 d
|
12.78±0.73 cd
|
7.82±0.31 f-jl
|
3.93±0.20 dj
|
8.60±0.89 ab
|
HR I |
16.70±0.90 ad
|
TS I |
10.03±1.10 d
|
0.68±0.02 g-i
|
14.71±1.13 de
|
6.80±0.49 eg
|
5.11±0.68 lm
|
12.00±2.45 c-k
|
HR J |
12.50±0.40 a
|
TS J |
9.17±0.56 d
|
0.63±0.02 fh
|
14.56±1.27 de
|
6.87±0.62 ei
|
3.83±0.18 cdi
|
11.40±0.89 bj
|
HR K |
65.00±4.40 j
|
TS K |
18.68±2.20 f
|
0.98±0.07 k
|
19.10±1.86 fg
|
5.24±0.10 bc |
3.00±0.10 b
|
13.33±1.15 g-l
|
HR L |
82.00±7.90 k
|
TS L |
4.06±0.88 ac
|
0.27±0.01 bc
|
14.86±1.33 de
|
6.73±0.42 ef |
3.81±0.29 cdh
|
11.67±0.58 bk
|
HR M |
22.20±3.40 dg
|
TS M |
5.17±0.19 bc
|
0.42±0.02 e
|
12.28±1.21 cd
|
8.14±0.81 jk |
5.27±0.15 m
|
9.75±0.96 bf
|
HR N |
23.30±1.60 e-g
|
TS N |
2.19±0.10 a
|
0.23±0.01 ac
|
9.53±0.75 ab
|
10.49±1.20 mn
|
3.62±0.05 cde
|
11.00±2.31 bi
|
HR O |
14.80±2.20 ab
|
TS O |
2.59±0.21 a
|
0.23±0.01 ab
|
11.38±0.94 bc
|
8.79±0.97 kl
|
3.71±0.56 cdf
|
9.40±1.14 bd
|
Table 4.
Phenylpropanoid production in Hypericum perforatum transgenic shoots.
Table 4.
Phenylpropanoid production in Hypericum perforatum transgenic shoots.
|
TP (mg GA·g-1 DW) |
TF (mg C·g-1 DW) |
TFA (mg C·g-1 DW) |
TPA (mg P·g-1 DW) |
TCT (mg CG·g-1 DW) |
PAL (pkat·mg-1 P) |
PPO (nkat·mg-1 P) |
NTS |
45.06±1.40 f
|
19.77±2.00 eg
|
5.16±0.21 f
|
3.60±0.02 h
|
3.30±0.02 hj
|
0.72±0.10 fg
|
5.60±0.26 a
|
TS A |
38.95±2.30 e
|
22.26±0.91 h
|
4.14±0.25 d
|
2.44±0.08 ef
|
2.85±0.14 g
|
2.80±0.30 i
|
51.39±3.01 l
|
TS B |
42.13±1.63 ef
|
18.09±1.09 de
|
4.64±0.01 e
|
2.38±0.18 ef
|
2.41±0.03 cf
|
0.63±0.25 eg
|
24.45±1.60 j
|
TS C |
61.05±3.29 h
|
26.14±1.38 i
|
5.80±0.20 g
|
3.61±0.16 h
|
3.17±0.16 i-k
|
0.30±0.11 b-d
|
51.17±2.44 l
|
TS D |
51.69±0.90 g
|
26.32±1.53 i
|
7.08±0.13 h
|
3.64±0.03 h
|
3.35±0.13 hk
|
0.70±0.19 fg
|
27.74±1.02 k
|
TS E |
62.03±0.74 h
|
22.86±1.03 h
|
7.56±0.11 i
|
3.85±0.28 i
|
3.47±0.23 h
|
0.16±0.01 ab
|
17.65±1.85 gh
|
TS F |
61.63±4.28 h
|
27.82±0.45 i
|
10.44±0.37 j
|
4.35±0.13 j
|
4.20±0.13 l
|
1.00±0.10 h
|
23.13±2.60 ij
|
TS G |
40.70±1.57 e
|
22.80±1.84 h
|
4.18±0.16 d
|
3.22±0.07 g
|
2.82±0.20 fg
|
0.24±0.00 ac
|
14.26±2.14 bf
|
TS H |
35.12±1.48 bd
|
19.26±0.97 ef
|
4.13±0.11 d
|
2.30±0.14 df
|
2.51±0.04 cd
|
0.35±0.02 b-d
|
13.61±0.42 bd
|
TS I |
32.09±0.16 b
|
16.95±1.03 cd
|
2.50±0.16 b
|
2.42±0.05 ef
|
2.06±0.09 b
|
0.59±0.06 ef
|
20.57±3.27 i
|
TS J |
54.22±3.44 g
|
23.53±1.89 h
|
5.42±0.22 f
|
3.49±0.02 h
|
3.28±0.11 hi
|
0.77±0.09 fg
|
11.89±0.06 b
|
TS K |
35.62±1.23 cd
|
15.18±0.19 bc
|
3.30±0.26 c
|
2.27±0.06 de
|
2.72±0.25 d-g
|
0.29±0.14 ad
|
15.32±0.76 c-fh
|
TS L |
39.36±4.03 e
|
20.64±1.48 fg
|
3.53±0.24 c
|
1.88±0.09 c
|
2.53±0.18 ce
|
0.10±0.01 a
|
15.18±0.37 c-g
|
TS M |
32.13±2.34 b
|
14.80±0.78 b
|
2.48±0.14 b
|
1.53±0.01 b
|
2.03±0.03 b
|
0.31±0.05 b-d
|
11.49±3.14 b
|
TS N |
35.17±0.08 bc
|
8.73±0.19 a
|
2.61±0.16 b
|
2.20±0.10 d
|
1.92±0.06 b
|
0.97±0.07 h
|
13.50±1.10 bc
|
TS O |
23.26±0.99 a
|
7.36±0.96 a |
1.85±0.03 a
|
1.29±0.18 a
|
1.37±0.08 a
|
0.45±0.04 de
|
14.22±0.01 be
|
Table 5.
Naphthodianthrone production in Hypericum perforatum transgenic shoots.
Table 5.
Naphthodianthrone production in Hypericum perforatum transgenic shoots.
|
HYP (µg·g-1 DW) |
PHYP (µg·g-1 DW) |
PPHYP (µg·g-1 DW) |
NTS |
28.55±1.45 g
|
660.64±35.33 b
|
91.92±1.12 a
|
TS A |
24.33±1.19 f
|
1101.39±20.57 e
|
366.51±11.85 f
|
TS B |
69.41±2.54 i
|
1915.34±56.88 g
|
477.90±3.41 h
|
TS C |
24.14±1.01 f
|
755.99±19.09 c
|
356.37±5.67 ef
|
TS D |
21.15±1.35 e
|
1096.43±35.96 de
|
364.90±10.08 f
|
TS E |
27.13±1.09 g
|
1543.25±43.12 f
|
441.51±8.61 g
|
TS F |
19.18±1.27 e
|
758.68±62.04 c
|
348.99±3.32 e
|
TS G |
23.98±0.95 f
|
1495.50±26.04 f
|
511.09±9.89 i
|
TS H |
34.63±1.11 h
|
1178.44±64.78 e
|
578.80±12.22 j
|
TS I |
13.25±0.56 b
|
626.12± 21.77 b
|
142.61±7.54 b
|
TS J |
14.16±1.22 bc
|
1062.12±9.88 d
|
220.77±3.12 d
|
TS K |
23.53±1.85 f
|
1074.68±12.99 de
|
198.98±6.09 c
|
TS L |
26.67±1.18 |
1463.86±38.31 f
|
506.73±12.49 i
|
TS M |
16.97±0.72 d |
652.18±8.76 b
|
147.43±2.67 b
|
TS N |
11.11±0.86 a
|
537.09±18.71 a
|
190.03±7.91 c
|
TS O |
15.87±0.58 cd
|
647.69±11.67 b
|
146.94±1.20 b
|
Table 6.
Antioxidant and radical scavenging activities in Hypericum perforatum transgenic shoots.
Table 6.
Antioxidant and radical scavenging activities in Hypericum perforatum transgenic shoots.
|
CUPRAC (μМ Т·g-1 DW) |
FRAP (μМ Fe2+·g-1 DW) |
ABTS (μМ Т·g-1 DW) |
LPI (%) |
H2O2 SA (%) |
O2•- SA (%) |
OH• SA (mM M·g-1 DW) |
NTS |
178.27±2.90 e-g
|
432.11±0.74 fg
|
123.51±2.90 i
|
74.10±2.40 dg
|
18.47±0.92 a
|
34.68±1.15 df
|
1.63±0.02 i
|
TS A |
188.20±4.83 g
|
492.19±14.87 h
|
113.54±4.05 h
|
66.53±1.92 c
|
26.36±0.64 dg
|
30.95±0.78 c
|
1.71±0.03 i
|
TS B |
186.66±4.11 g
|
440.00±21.21 g
|
100.89±1.89 g
|
60.60±0.32 b
|
28.87±2.30 hikl
|
38.15±0.48 gj
|
1.53±0.05 d-h
|
TS C |
210.44±10.62 h
|
530.79±6.39 i
|
123.21±2.53 i
|
84.72±0.52 ij
|
22.42±1.50 bc
|
33.42±1.40 d
|
1.44±0.00 cd
|
TS D |
212.95±11.95 h
|
550.13±28.47 i
|
144.42±2.21 j
|
75.89±4.12 e-g
|
25.56±1.30 de
|
27.27±0.80 b
|
1.70±0.05 i
|
TS E |
236.27±10.40 i
|
595.79±20.97 j
|
142.19±1.58 j
|
71.97±4.12 d
|
27.86±0.64 dh-jmn
|
33.39±2.43 d
|
1.71±0.01 i
|
TS F |
250.81±14.52 i
|
649.91±37.46 k
|
168.75±0.00 k
|
63.97±4.08 c
|
27.46±1.06 e-gi
|
41.94±2.23 k
|
1.85±0.05 j
|
TS G |
166.52±8.81 de
|
431.05±9.86 g
|
98.66±2.23 e-g
|
84.97±0.48 ij
|
27.11±2.00 e-h
|
34.41±0.36 de
|
1.27±0.06 b
|
TS H |
157.86±3.44 cd
|
390.79±14.89 ce
|
88.69±6.70 d
|
82.69±1.29 hi
|
25.58±0.31 df
|
26.60±0.10 b
|
1.45±0.04 cf
|
TS I |
126.78±7.50 b
|
366.93±20.15 c
|
78.87±7.16 bc
|
79.97±0.77 h
|
28.76±2.19 gjk
|
24.20±3.51 a
|
1.50±0.00 ch
|
TS J |
202.22±4.36 h
|
586.18±1.67 j
|
92.19±10.00 df
|
51.50±2.61 a
|
24.46±1.77 cd
|
37.94±1.52 gi
|
1.73±0.03 i
|
TS K |
152.95±4.85 c
|
380.26±8.66 cd
|
84.97±5.65 cd
|
91.33±3.01 k
|
33.15±0.35 o
|
36.12±0.57 e-g
|
1.48±0.01 cg
|
TS L |
168.69±4.36 df
|
401.32±49.87 d-f
|
90.63±1.89 de
|
63.76±3.13 c
|
21.92±1.13 b
|
38.11±0.12 gh
|
1.39±0.10 c
|
TS M |
131.96±1.88 b
|
328.42±11.16 b
|
76.12±4.10 b
|
83.00±4.73 hj
|
30.00±0.71 k-m
|
40.17±0.39 hjk
|
1.45±0.03 ce
|
TS N |
133.68±9.34 b
|
368.68±13.40 c
|
83.63±0.68 bd
|
74.04±1.43 df
|
30.10±0.42 kln
|
57.94±1.33 l
|
1.24±0.09 b
|
TS O |
79.56±6.05 a
|
230.88±13.41 a
|
48.66±0.63 a
|
73.03±1.17 de
|
28.81±0.44 gjl
|
39.67±2.53 h-j
|
1.14±0.02 a
|
Table 7.
Antioxidant enzymes and oxidative stress markers in Hypericum perforatum transgenic shoots.
Table 7.
Antioxidant enzymes and oxidative stress markers in Hypericum perforatum transgenic shoots.
|
PX (nkat·mg-1 P) |
АPX (pkat·mg-1 P) |
CAT (nkat·mg-1 P) |
SOD (U·mg-1 P) |
H2O2 (μM·g-1 FW) |
O2•- (nM·min-1·g-1 FW) |
MDA (nM·g-1 FW) |
NTS |
0.03±0.00 a
|
66.15±10.54 j
|
0.80±0.10 j
|
2.05±0.20 j
|
1.34±0.04 j
|
0.64±0.10 efh
|
1.42±0.08 j
|
TS A |
0.14±0.02 gk
|
104.96±7.42 k
|
0.48±0.00 h
|
2.44±0.16 k
|
0.38±0.06 a
|
0.35±0.04 b
|
0.41±0.02 a
|
TS B |
0.29±0.01 m
|
34.08±2.71 d-h
|
0.55±0.01 i
|
1.19±0.23 hi
|
0.69±0.02 b
|
0.67±0.01 ghj
|
0.82±0.02 bd
|
TS C |
0.09±0.02 bd
|
124.69±12.61 l
|
1.02±0.02 k
|
7.51±0.47 l
|
0.63±0.03 b
|
0.24±0.02 a
|
1.18±0.02 g-i
|
TS D |
0.14±0.03 fgi
|
28.32±2.06 cg
|
0.87±0.02 j
|
1.32±0.32 i
|
0.81±0.08 c
|
0.44±0.04 c
|
1.07±0.01 fg
|
TS E |
0.15±0.00 h-k
|
32.08±1.45 d-g
|
0.51±0.07 hi
|
0.84±0.10 b-df
|
1.04±0.08 h
|
0.59±0.08 dfi
|
1.37±0.14 j
|
TS F |
0.20±0.03 l
|
25.96±3.66 cd
|
0.19±0.03 c
|
1.28±0.15 i
|
0.92±0.06 d-f
|
0.57±0.02 de
|
1.10±0.05 fi
|
TS G |
0.08±0.01 bc
|
41.52±5.05 hi
|
1.17±0.10 l
|
0.75±0.07 b-d
|
0.87±0.08 ce
|
0.55±0.02 d
|
0.74±0.09 b
|
TS H |
0.10±0.03 be
|
17.10±3.68 ab
|
0.33±0.03 f
|
0.47±0.15 a
|
0.64±0.02 b
|
0.62±0.03 e-g
|
0.90±0.01 c-e
|
TS I |
0.11±0.00 deg
|
27.93±0.26 cf
|
0.27±0.02 de
|
0.84±0.05 b-dg
|
0.95±0.05 e-g
|
0.75±0.06 k
|
1.45±0.01 j
|
TS J |
0.13±0.02 f-h
|
20.05±0.78 ac
|
0.11±0.00 a
|
0.60±0.04 ab
|
1.18±0.06 i
|
1.22±0.03 n
|
1.75±0.10 k
|
TS K |
0.08±0.02 b
|
21.73±3.46 bc
|
0.40±0.01 g
|
0.73±0.03 b-d
|
1.17±0.01 i
|
0.65±0.04 g-i
|
0.77±0.03 bc
|
TS L |
0.11±0.01 c-f
|
26.73±1.30 ce
|
0.18±0.02 bc
|
0.69±0.02 ad
|
0.88±0.07 cf
|
0.83±0.06 l
|
0.85±0.04 be
|
TS M |
0.05±0.01 a
|
45.48±3.36 i
|
0.23±0.05 ce
|
0.80±0.00 b-e
|
0.84±0.08 cd
|
0.73±0.03 jk
|
1.08±0.05 fh
|
TS N |
0.14±0.01 fgj
|
73.75±0.67 j
|
0.12±0.01 ab
|
1.02±0.06 e-h
|
1.02±0.06 gh
|
0.45±0.08 c
|
1.39±0.10 j
|
TS O |
0.04±0.01 a
|
13.06±2.41 a
|
0.22±0.00 cd
|
0.66±0.02 ac
|
1.06±0.03 h
|
0.95±0.07 m
|
0.97±0.10 ef
|