1. Introduction
Many RNA molecules are produced in eukaryotic cells, but only a minute fraction of the total RNA is converted into protein, and others devoid of protein-coding regions are called non-coding RNAs (ncRNAs). While the small ncRNAs (sncRNAs) such as microRNA (miRNA), small nuclear RNA (snRNA), small interfering RNA (siRNA), and PIWI-interacting RNAs (piRNAs) have a size <200 nucleotides, whereas the long ncRNAs (LncRNAs) have a length of >200 bp [
1,
2]. The LncRNAs have been found to play a crucial role in regulating gene expressions in various physiological, pathological, and immunological processes in higher organisms [
3]. In insects, they play a key role in the regulation of the developmental (sex-determination, immunity, and morphogenesis) as well as behavioral (sleeping, foraging, courtship, etc) processes [
2,
4,
5]. However, studies on insect LncRNAs are mostly limited to model species [
2]. With the advancement of high throughput technology (HTS), many insects, including the nonmodal species, have been characterized and identified several novel non-coding RNAs (ncRNAs). For example, several novel LncRNAs have been identified in
P. xylostella that play important roles in developing host resistance by acting as a precursor molecule for the production of miRNAs. The lnc-GSTu1-AS has been reported in chlorantraniliprole-resistant strains of
P. xylostella, and its deletion significantly decreased the host resistance to chlorantraniliprole. It has been reported that the lnc-GSTu1-AS affects the expression of GSTu1 by targeting miR-8525-5p, leading to enhanced resistance to chlorantraniliprole [
6]. The function of microRNAs (miRNAs) is mainly involved in the regulation of the genes' expression through binding on the mRNAs and modulates metabolism, immunity, and developmental processes via post-transcriptional regulation [
7,
8]. Synthetic miRNA mimics and inhibitors has been identified that target key genes and interfere with the phenotypic expression of the insect [
9,
10,
11,
12]. Although several miRNAs have been reported to have possible involvement in regulating immune-related processes, mechanistic studies on their role in insect immunity are largely limited [
13].
The innate immunity of insects comprises cellular and humoral responses [
14,
15]. Cellular immunity primarily refers to phagocytosis and encapsulation by the hemocytes [
16], while humoral immunity deals with the production of antimicrobial peptides [
17] in the fat body and release rapidly into the hemolymph, leading to the activation of the prophenoloxidase (proPO) or the melanization cascade [
18,
19,
20]. proPO is activated via serine proteases (SPs) cascades when insect pattern recognition receptors (PRRs) recognize the pathogen-associated molecular patterns (PAMPs). This activates of prophenoloxidase-activating proteinase (PAP) [
21]. The PO cascade plays a crucial role during the fungal invasion by converting phenols to quinones and subsequently polymerizing to form melanin to defend against pathogens [
22]. PAPs are the direct activator of proPO, while Serpins can prevent the SPs from excessively regulating melanization [
23,
24]. In addition to the SPs cascades, PAPs and PO activities are also regulated by miRNAs [
25].
P. xylostella (Linnaeus) (Lepidoptera: Plutellidae) causes significant economic losses to cruciferous crops [
26,
27]. The present control strategy mainly involves the application of wide-spectrum insecticides, resulting in the development of resistance and environmental and health risks [
28,
29]. Entomopathogenic fungal insecticides are promising pest management tools that attach to the cuticle, germinate, and penetrate hemolymph, leading to host death [
30,
31,
32]. For example, members under the genus
Beauveria and
Metarhizium have been successfully applied against
Aedes albopictus (Skuse) [
33],
Bemisia tabaci (Gennadius) [
34],
Spodoptera frugiperda Smith [
35] and
P. xylostella [
36]. However, insects respond to pathogenic infections by activating their innate immune responses that develop resistance against EPF [
37]. The miRNAs play an essential role in regulating multiple vital biological processes (development, immunity, insecticide resistance, metamorphosis, reproduction etc.) of insects. Since miRNAs and their binding site sequences are target-specific, their application allows the killing of specific pests without interfering with non-target organisms. Application of miRNA-based pest control strategy can reduce the use of hazardous pesticides and associated resistant strain development and environmental pollution. Moreover, artificial regulation of the activity of specific miRNAs can help restore insecticide sensitivity so that existing pesticides can work effectively [
38]. Therefore, miRNA-based strategy could be the key to formulating a new generation of effective, environment-friendly pest control agents [
39]. In our previous study, three PAPs (PAP1, PAP3, and PAP3a) were identified in
P. xylostella [
40], and based on transcriptome data we predicted that non-coding RNAs might regulate them. Here, we aim to delineate the interaction mechanism between LncRNA and miRNA of
P. xylostella infected with
Metarhizium anisopliae, using in vitro and in vivo experiments. The identified candidate miRNA pxy-miR-965-5p that targets PAP1 could serve as a promising candidate for pest management strategies.
3. Discussion
Insects solely depend on the innate immune system for recognizing and targeting foreign particles since they don’t possess adaptive immunity, and the innate immunity largely depends on prophenoloxidase, which plays a key role in the cellular and humoral defense of insects [
14,
17,
41]. The proPO system is strongly regulated by the different serine proteases such as PAPs, Serpins, and SPs/ Serine protease homologs (SPs/ SPHs). PAPs release active proPO at specific sites, mediating melanization. Although PO-mediated melanization can kill invading pathogens, overproduction of the intermediates can harm the insects. Serpin can prevent excessive melanism by inhibiting SPs/ SPHs activity and blocking proPO activation. SPs/ SPHs and Serpin rigorously regulate the activation of proPO in time and space, removing the invading exogenous substances and evading the detrimental effects of intermediates [
42,
43,
44,
45,
46,
47]. Therefore, the PO cascade is a good target for biological pest control. However, so far, the immune response to pathogens and the regulatory mechanism of PO production has only been preliminarily described at the protein level in some model insects, and the precise regulation of PO-mediated melanism is still not understood [
45,
48].
It has been described that the expression of Serpin-2, Serpin-4, Serpin-5 [
49], PxSP1, PxSP2, PPO1, and PPO2 [
50] were regulated after pathogenic infection in
P. xylostella which triggered the PO activity. Similarly, an increased PO activity was reported in hemolymph at 24 - 48 hpi by
M. anisopliae [
49]. In the present study the PO activity increased in the plasma of
P. xylostella at 12 to 36 h after
M. anisopliae infection, (
Figure 1C). The high PO activity may be due to the intense immune response in the early stages of infection that gradually declines as the infection progresses. Previous experiments showed that PPAF-II acted as a module for PO binding through the central cleft in
Holotrichia Diomphalia Bates [
51], and PAP-1, PAP-2, PAP-3 showed diverse abilities to activate PO activity in
Manduca sexta (Linnaeus) [
52,
53,
54,
55]. Correspondingly, in this study, PAP1 activity was compared to trypsin activity (
Figure 2A and
2C), and the activated PAP1 could activate the PO activity in
P. xylostella (
Figure 2C).
miRNAs targeted gene expression may play vital roles at the post-transcriptional level in maintaining homeostasis and plasticity of immunity. miRNA mimics or inhibitors were used to assess the function of miRNAs in insects [
56,
57]. For example, in Drosophila, bioinformatics was used to detect potential miRNA targets and express the profiles of miRNAs involved in regulating immune response [
58]. miR-8 has been reported to target the transcripts of GNBP3 and then negatively regulate the expression of Drosomycin and Diptericin in Drosophila. It also indicated that the transcript levels of the AMPs were significantly elevated in the absence of the miR-8 in Drosophila larvae and flies [
59]. The bioinformatic analysis predicted that 7 miRNAs might participate in immunity by targeting PAP1 in post
M. anisopliae infection (
Figure 3A). The results of the dual luciferase assay showed that only pxy-miR-279a-3p and pxy-miR-965-5p could significantly reduce the expression level of the PAP1 in 293T cells (
Figure 3B).
The seed region (g2-g8) of miRNA is crucial for target recognition, and the mutations of seed sequence may disrupt the interaction between miRNAs and targeted mRNAs [
60,
61,
62]. Experiments have depicted that the mismatches in positions 2–7 of miR-7 and miR-278 strongly reduced the magnitude of target regulation in cells [
63]. The targeted site of let-7a mismatched at positions 3 and 4 or mismatched at positions 5 and 6 would reduce the repression effect on targeted mRNA in HeLa cells [
64]. It also indicated that the ΔG value of this interaction is an important determinant of activity [
65]. The findings of the current study proved that the targeted site in the seed region of pxy-miR-965-5p mismatched at positions 4 or 6, leading to a loss of ability to suppress PAP1 (
Figure S1). Moreover, recent research also showed their involvement in recognition and regulation [
65]. The mutations of a single nucleotide at g11–g16 lead to functional defects of miRNA let-7a [
66].
Fungal infection can alter the expression of insect miRNAs [
67]. For instance, pathogen infection leads to the upregulation of miR-92, which plays a major role in the developmental process of
A. albopictus and
Culex quinquefasciatus (Say) [
68]. In the present study, an upregulated expression of pxy-miR-965-5p was observed upon
M. anisopliae infection. The expression patterns of PAP1 and pxy-miR-965-5p after
M. anisopliae infection appeared to exhibit a negative regulatory trend (
Figure 4B). In addition, the tissue expression patterns of pxy-miR-279a-3p and pxy-miR-965-5p were similar to PAP1, which was upregulated in the epidermis and fat body (
Figure 4A). Generally, miRNAs function by down-regulating the expression of targeted genes [
69], and the expression pattern of most miRNAs and their targeted mRNAs had spatial-temporal specificity [
70,
71]. miR-92b can down-regulate Mef2 and were expressed in the heart and specific muscles in Drosophila [
72]; miR-927 can down-regulate Kr-h1 in the fat body of Drosophila [
73]; miR-184 can down-regulate the CYP303A1 in the integument of
L. migratoria [
74].
The expression of EcR was down-regulated by miR-34-5p in
Helicoverpa armigera (Hübner), Spodoptera exigua Hübner, and
P. xylostella, but the physiological effects were not quite the same [
75]. JNK, regulated by miRNA-184, can regulate the ROS metabolism and PO activity but was not exactly a simple corresponding relationship in vivo in the agomir injection experiments [
76]. We observed that PAP1 expression was down-regulated after the pxy-miR-965-5p mimic was injected into
P. xylostella, leading to decreased PO activity; on the control, pxy-miR-965-5p inhibitor was injected into
P. xylostella, leading to increased PO activity (
Figure 5A,
Figure 6B). The pathogen's infection would increase with the repression of the PO cascade. A repressed PO cascade increased the mortality of
L. migratoria nymphs during
M. anisopliae infection [
77]. The activity of PO cascade can block baculovirus infection, but the inhibition can be rescued by serpin-9 [
78]. The results of our study demonstrated that pxy-miR-965-5p could repress the PO cascade. It indicated that the overexpression of pxy-miR-965-5p was conducive to
M. anisopliae infection (
Figure 6A). Therefore, during
M. anisopliae infection, pxy-miR-965-5p was upregulated, and the PO cascade was suppressed, resulting in increased mortality in
P. xylostella (
Figure 6).
LncRNA is a non-coding single-stranded RNA (>200 bp) composed of exon or intron sequences. It can be clipped, capped, polyadenylate, and can play a regulatory role in the nucleus or cytoplasm [
79]. They are involved in regulating the gene expression in the nucleus via controlling the local chromatin structure or recruiting regulatory factors to specific sites, such as acting as enhancers to regulate gene expression [
80,
81]. LncRNA regulates gene expression by altering the scaffold activity of chromatin modification proteins such as methyltransferase, demethylase, acetyltransferase, and deacetylase. And recruit these proteins to target sites by either cis-regulation (regulating transcription of nearby genes) or trans-regulation (regulating transcription of genes farther away in the genome) [
82]. In the cytoplasm, LncRNA interacts with other types of RNA, affecting mRNA stability, mRNA translation and acting as a miRNA sponge for miRNA absorption [
83]. miR-2834 can reduce the expression of vitellogenin (vg) in silkworms, thereby affecting the formation of eggs, while lncR26319 can act as a miRNA sponge to reduce miR-2834's silting of vg, thereby increasing vg expression [
84]. At present, the functional research of LncRNA mainly focuses on the knockdown. The mechanisms of dsRNA and ASO are different. dsRNA is cleaved by Dicer enzyme and then combined with AGO to form RISC silencing complex, which is then complementary and paired with target RNA to perform functions, while ASO can directly bind to target RNA. The RNase-H enzyme is recruited in the nucleus or cytoplasm to cut the target RNA to perform the function [
85]. In the present study, based on the whole transcriptome sequencing and dual-luciferase assays, four LncRNAs, MSTRG.32910.1, MSTRG.7100.1, MSTRG.6802.1, and MSTRG.22113.1 were identified (
Figure S2). In vitro, we tested the activity of four LncRNAs by dual-luciferase (
Figure 7). In vivo, we silenced these four LncRNAs through ASO injection. The results demonstrated that ASO injection can effectively silence four LncRNAs (
Figure 8A). When MSTRG.7100.1 and MSTRG.22113.1 were silenced, the expression of pxy-miR-965-5p correspondingly increased. However, silence the MSTRG.32910.1 and MSTRG.6802.1 fails to alter the expression of pxy-miR-965-5p (
Figure 8B).
Author Contributions
Conceptualization, X.X., F.J.; methodology, Z.Z., J.H., L.Z.; validation, Z.Z.; formal analysis, Z.Z., F.J., J.Z.; resources, X.X., F.J.; writing—original draft preparation, Z.Z., X.X., F.J.; writing—review and editing, Z.Z., X.X., F.J., J.Z., S.D.M.; supervision, F.J., X.X.; funding acquisition, F.J. and X.X. All authors have read and agreed to the published version of the manuscript.
Figure 1.
The infection of P. xylostella by M. anisopliae. (A) The phenomena of infection. (B) The black patch in the integument of P. xylostella. (C) Determination of PO activity. “Control” indicates the group treated by conidia germinating medium, and “Ma” indicates the group infected by M. anisopliae. The column represented the PO activity, and data in the figure are mean ± SE (LSD and Duncan analysis: α = 0.05, n=3, letters on the columns indicate significant differences among groups, p < 0.05).
Figure 1.
The infection of P. xylostella by M. anisopliae. (A) The phenomena of infection. (B) The black patch in the integument of P. xylostella. (C) Determination of PO activity. “Control” indicates the group treated by conidia germinating medium, and “Ma” indicates the group infected by M. anisopliae. The column represented the PO activity, and data in the figure are mean ± SE (LSD and Duncan analysis: α = 0.05, n=3, letters on the columns indicate significant differences among groups, p < 0.05).
Figure 2.
PAP1 activated P. xylostella melanization in vitro. (A) The mode of PAP1 and IEGR mutant PAP1Xa. (B). The cleavage of PAP1Xa by Factor Xa, monoclonal antibody 6×His was used as the primary antibody, marker was on the left; protein PAP1Xa was on the line 57.3 kDa and protein activated PAP1Xa after cleaved was on line 29.4 kDa. (C) The enzyme activity of PAP1. The column represented the enzyme activity, data in the figure are mean ± SE (LSD and Duncan analysis: α = 0.05, n=3, letters on the columns indicate significant differences among groups, p < 0.05).
Figure 2.
PAP1 activated P. xylostella melanization in vitro. (A) The mode of PAP1 and IEGR mutant PAP1Xa. (B). The cleavage of PAP1Xa by Factor Xa, monoclonal antibody 6×His was used as the primary antibody, marker was on the left; protein PAP1Xa was on the line 57.3 kDa and protein activated PAP1Xa after cleaved was on line 29.4 kDa. (C) The enzyme activity of PAP1. The column represented the enzyme activity, data in the figure are mean ± SE (LSD and Duncan analysis: α = 0.05, n=3, letters on the columns indicate significant differences among groups, p < 0.05).
Figure 3.
The miRNA target prediction and verification of PAP1. (A) Sites on PAP1 targeted by miRNAs. 5’ UTR, 5’ Untranslated Region; ORF, Open Reading Frame; 3’ UTR, 3’ Untranslated Region. (B) The dual luciferase reporter assay of miRNAs to PAP1. The column represented the RFU (hRluc/hluc+) fold change of miRNA mimic treatment groups compared to negative control groups, data in the figure are mean ± SE (Two-tailed Student’s t-tests: α = 0.05, n=3, asterisks indicated significant differences between treatment and control groups, *p < 0.05, **p < 0.01, ***p < 0.001, NS = no significant differences).
Figure 3.
The miRNA target prediction and verification of PAP1. (A) Sites on PAP1 targeted by miRNAs. 5’ UTR, 5’ Untranslated Region; ORF, Open Reading Frame; 3’ UTR, 3’ Untranslated Region. (B) The dual luciferase reporter assay of miRNAs to PAP1. The column represented the RFU (hRluc/hluc+) fold change of miRNA mimic treatment groups compared to negative control groups, data in the figure are mean ± SE (Two-tailed Student’s t-tests: α = 0.05, n=3, asterisks indicated significant differences between treatment and control groups, *p < 0.05, **p < 0.01, ***p < 0.001, NS = no significant differences).
Figure 4.
The expression patterns of PAP1 and miRNA. (A) The expression patterns in different tissues. The column represented the relative expression of PAP1, pxy-miR-965-5p, and pxy-miR-279a-3p, data in the figure are mean ± SE (LSD and Duncan analysis: α = 0.05, n=3, letters on the columns indicated significant differences among different tissues, p < 0.05). (B) The expression patterns after M. anisopliae infection. The column represented the relative expression fold change of treatment groups compared to control groups; data in the figure are mean ± SE (Two-tailed Student’s t-tests: α = 0.05, n=3, asterisks indicated significant differences between treatment and control groups, *p < 0.05, **p < 0.01, ***p < 0.001, NS = no significant differences).
Figure 4.
The expression patterns of PAP1 and miRNA. (A) The expression patterns in different tissues. The column represented the relative expression of PAP1, pxy-miR-965-5p, and pxy-miR-279a-3p, data in the figure are mean ± SE (LSD and Duncan analysis: α = 0.05, n=3, letters on the columns indicated significant differences among different tissues, p < 0.05). (B) The expression patterns after M. anisopliae infection. The column represented the relative expression fold change of treatment groups compared to control groups; data in the figure are mean ± SE (Two-tailed Student’s t-tests: α = 0.05, n=3, asterisks indicated significant differences between treatment and control groups, *p < 0.05, **p < 0.01, ***p < 0.001, NS = no significant differences).
Figure 5.
The expression of PAP1 in P. xylostella under miRNA treatments. (A) The expression of PAP1 under pxy-miR-965-5p treatments. Ma, the group infected by M. anisopliae; Ma + NC, the group infected by M. anisopliae after mimics negative control injection; Ma + mimic, the group infected by M. anisopliae after pxy-miR-965-5p mimic injection; Ma + inhibitor, the group infected by M. anisopliae after pxy-miR-965-5p inhibitor injection. The column represented the relative expression of PAP1, data in the figure are mean ± SE (LSD and Duncan analysis: α = 0.05, n=3, letters on the columns indicate significant differences among groups, p < 0.05). (B) The expression of PAP1 under pxy-miR-279a-3p treatments. Ma, the group infected by M. anisopliae; Ma + NC, the group infected by M. anisopliae after mimics negative control injection; Ma + mimic, the group infected by M. anisopliae after pxy-miR-279a-3p mimic injection; Ma + inhibitor, the group infected by M. anisopliae after pxy-miR-279a-3p inhibitor injection. The column represented the relative expression of PAP1, data in the figure are mean ± SE (LSD and Duncan analysis: α = 0.05, n=3, letters on the columns indicate significant differences among groups, p < 0.05).
Figure 5.
The expression of PAP1 in P. xylostella under miRNA treatments. (A) The expression of PAP1 under pxy-miR-965-5p treatments. Ma, the group infected by M. anisopliae; Ma + NC, the group infected by M. anisopliae after mimics negative control injection; Ma + mimic, the group infected by M. anisopliae after pxy-miR-965-5p mimic injection; Ma + inhibitor, the group infected by M. anisopliae after pxy-miR-965-5p inhibitor injection. The column represented the relative expression of PAP1, data in the figure are mean ± SE (LSD and Duncan analysis: α = 0.05, n=3, letters on the columns indicate significant differences among groups, p < 0.05). (B) The expression of PAP1 under pxy-miR-279a-3p treatments. Ma, the group infected by M. anisopliae; Ma + NC, the group infected by M. anisopliae after mimics negative control injection; Ma + mimic, the group infected by M. anisopliae after pxy-miR-279a-3p mimic injection; Ma + inhibitor, the group infected by M. anisopliae after pxy-miR-279a-3p inhibitor injection. The column represented the relative expression of PAP1, data in the figure are mean ± SE (LSD and Duncan analysis: α = 0.05, n=3, letters on the columns indicate significant differences among groups, p < 0.05).
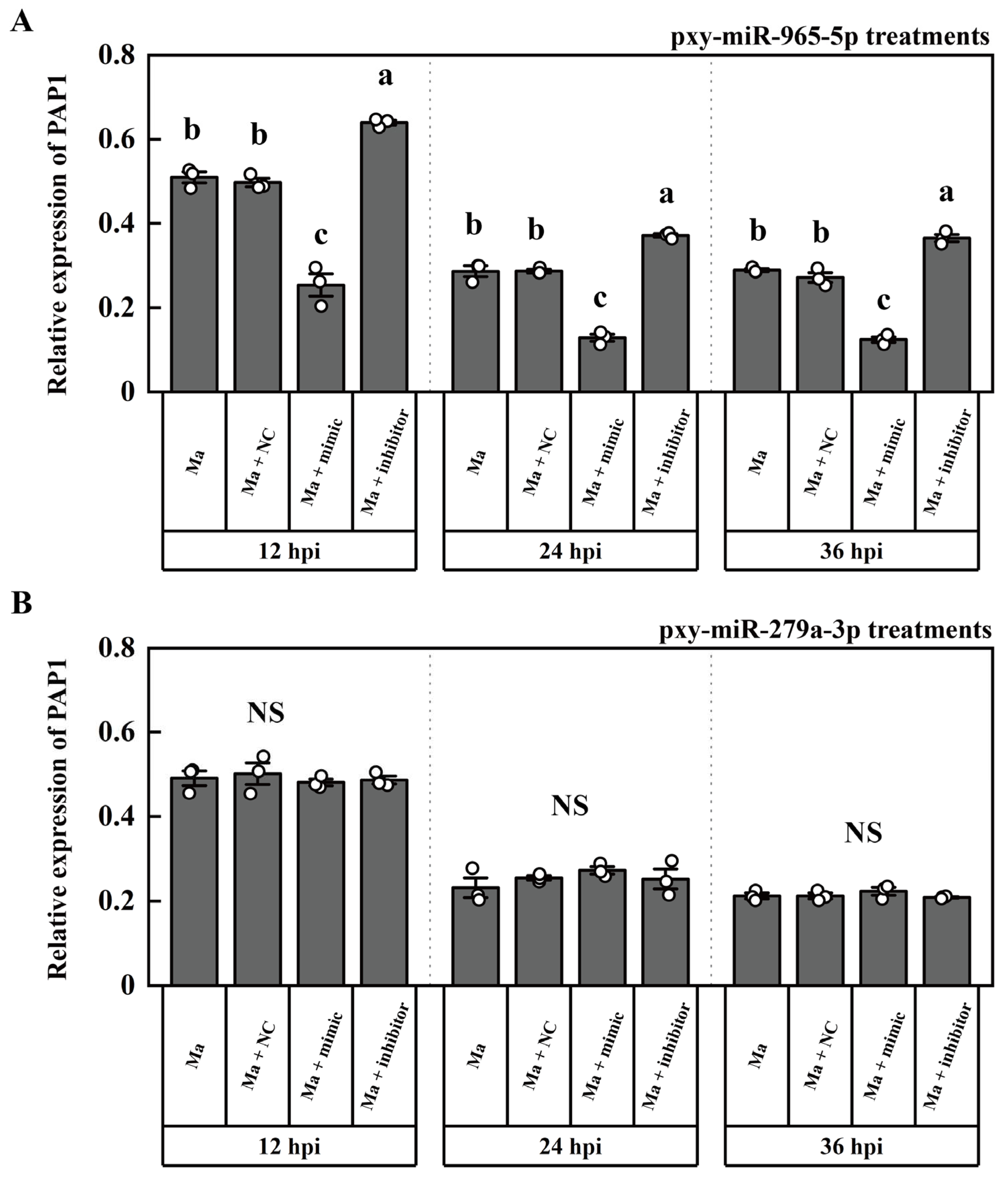
Figure 6.
Survival rates and PO activity of P. xylostella under miRNA treatments. (A) Survival rates of P. xylostella under miRNA treatments. Ma, the group infected by M. anisopliae; Ma + NC, the group infected by M. anisopliae after mimics negative control injection; Ma + pxy-miR-965-5p mimic, the group infected by M. anisopliae after pxy-miR-965-5p mimic injection; Ma + pxy-miR-965-5p inhibitor, the group infected by M. anisopliae after pxy-miR-965-5p inhibitor injection. The curve represented the survival rates (Log-rank test: α = 0.05, n=100, letters on the columns indicate significant differences among groups, p < 0.05). (B) PO activity of P. xylostella under miRNA treatments. (B) PO activity of P. xylostella under miRNA treatments. Ma, the group infected by M. anisopliae; Ma + NC, the group infected by M. anisopliae after mimics negative control injection; Ma + mimic, the group infected by M. anisopliae after pxy-miR-965-5p mimic injection; Ma + inhibitor, the group infected by M. anisopliae after pxy-miR-965-5p inhibitor injection. The column represented the PO activity, data in the figure are mean ± SE (LSD and Duncan analysis: α = 0.05, n=3, letters on the columns indicate significant differences among groups, p < 0.05).
Figure 6.
Survival rates and PO activity of P. xylostella under miRNA treatments. (A) Survival rates of P. xylostella under miRNA treatments. Ma, the group infected by M. anisopliae; Ma + NC, the group infected by M. anisopliae after mimics negative control injection; Ma + pxy-miR-965-5p mimic, the group infected by M. anisopliae after pxy-miR-965-5p mimic injection; Ma + pxy-miR-965-5p inhibitor, the group infected by M. anisopliae after pxy-miR-965-5p inhibitor injection. The curve represented the survival rates (Log-rank test: α = 0.05, n=100, letters on the columns indicate significant differences among groups, p < 0.05). (B) PO activity of P. xylostella under miRNA treatments. (B) PO activity of P. xylostella under miRNA treatments. Ma, the group infected by M. anisopliae; Ma + NC, the group infected by M. anisopliae after mimics negative control injection; Ma + mimic, the group infected by M. anisopliae after pxy-miR-965-5p mimic injection; Ma + inhibitor, the group infected by M. anisopliae after pxy-miR-965-5p inhibitor injection. The column represented the PO activity, data in the figure are mean ± SE (LSD and Duncan analysis: α = 0.05, n=3, letters on the columns indicate significant differences among groups, p < 0.05).
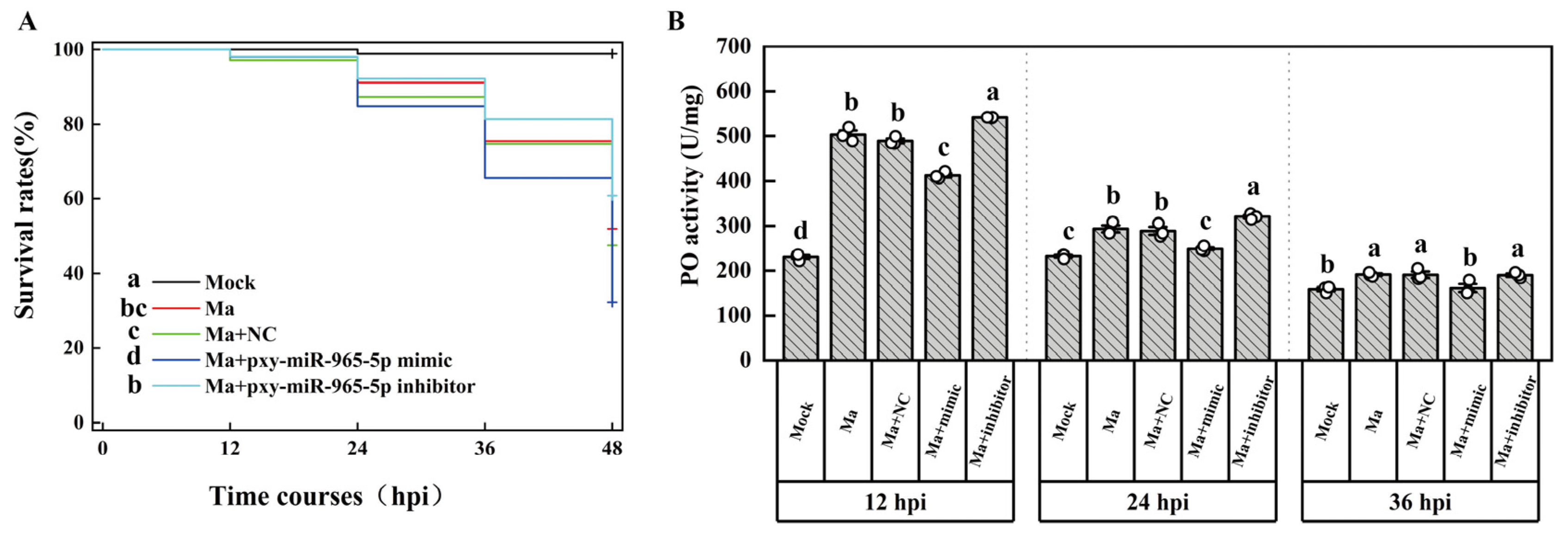
Figure 7.
The dual luciferase reporter assay of LncRNA to pxy-miR-965-5p. The column represented the RFU (hRluc/hluc+) fold change of miRNA mimic treatment groups compared to negative control groups, data in the figure are mean ± SE (Two-tailed Student’s t-tests: α = 0.05, n=3, asterisks indicated significant differences between treatment and control groups, **p < 0.01).
Figure 7.
The dual luciferase reporter assay of LncRNA to pxy-miR-965-5p. The column represented the RFU (hRluc/hluc+) fold change of miRNA mimic treatment groups compared to negative control groups, data in the figure are mean ± SE (Two-tailed Student’s t-tests: α = 0.05, n=3, asterisks indicated significant differences between treatment and control groups, **p < 0.01).
Figure 8.
The expression of LncRNA and miRNA in P. xylostella after ASO injection. (A) The expression of LncRNA after ASO injection. Ma, the group infected by M. anisopliae; Ma + NC, the group infected by M. anisopliae after ASO negative control injection; Ma + ASO, the group infected by M. anisopliae after ASO injection. The column represented the relative expression of LncRNA; data in figure are mean ± SE (LSD and Duncan analysis: α = 0.05, n=3, letters on the columns indicate significant differences among groups, p < 0.05). (B) The expression of pxy-miR-965-5p after ASO injection. Ma, the group infected by M. anisopliae; Ma + NC, the group infected by M. anisopliae after ASO negative control injection; Ma + ASO, the group infected by M. anisopliae after ASO injection. The column represented the relative expression of LncRNA; data in the figure are mean ± SE (LSD and Duncan analysis: α= 0.05, n=3, letters on the columns indicate significant differences among groups, p < 0.05).
Figure 8.
The expression of LncRNA and miRNA in P. xylostella after ASO injection. (A) The expression of LncRNA after ASO injection. Ma, the group infected by M. anisopliae; Ma + NC, the group infected by M. anisopliae after ASO negative control injection; Ma + ASO, the group infected by M. anisopliae after ASO injection. The column represented the relative expression of LncRNA; data in figure are mean ± SE (LSD and Duncan analysis: α = 0.05, n=3, letters on the columns indicate significant differences among groups, p < 0.05). (B) The expression of pxy-miR-965-5p after ASO injection. Ma, the group infected by M. anisopliae; Ma + NC, the group infected by M. anisopliae after ASO negative control injection; Ma + ASO, the group infected by M. anisopliae after ASO injection. The column represented the relative expression of LncRNA; data in the figure are mean ± SE (LSD and Duncan analysis: α= 0.05, n=3, letters on the columns indicate significant differences among groups, p < 0.05).