1. Introduction
Acute myeloid leukemia (AML) is one of the most aggressive hematologic malignancies, primarily targeting older adults aged ≥ 65. It is characterized by genetic and epigenetic defects that block the development of myeloid progenitor cells in the bone marrow at early stages of differentiation and promote the uncontrolled growth of leukemic blasts. Combination chemotherapy with cytosine β-D-arabinofuranoside (cytarabine) and an anthracycline antibiotic (e.g., daunorubicin) has been the frontline treatment for AML for more than 40 years and is relatively successful for younger patients. However, older individuals are mainly unfit for intensive chemotherapy and their treatment options remain sparse, resulting in a very short median overall survival (6-25 months) [
1,
2]. Although several novel targeted AML drugs are currently available, their impact on long-term patient survival is yet to be determined [
1,
3,
4]. Since maturation block is the primary feature of AML blasts, differentiation therapy presents an attractive alternative option for treating this disease [
5]. One AML subtype, acute promyelocytic leukemia (APL), has been successfully treated with the combination of the natural differentiation inducer all-
trans-retinoic acid (ATRA) and arsenic trioxide [
6]. However, no differentiation therapy is currently available for non-APL AML.
The hormonal form of vitamins D
3 and D
2, 1α,25-dihydroxyvitamin D
3/D
2 (1,25D
3/2), is a well-known inducer of myeloid differentiation in various non-APL AML cell types [
7,
8]. 1,25D
3 is the physiological ligand of the vitamin D receptor (VDR), a member of the nuclear receptor subfamily type II that includes retinoid X receptors (RXRs) and retinoic acid receptors. Upon binding 1,25D
3, VDR interacts with RXRα and/or other transcription factors, such as purine-rich box-1 (PU.1) or CCAAT enhancer binding protein alpha (CEBPα), to form protein complexes that act as ligand-activated transcription factors [
9,
10].
A major obstacle to the clinical development of vitamin D derivatives (VDDs) - 1,25D
3/2 and their synthetic analogs - for AML therapy is their dose-limiting calcemic toxicity. Clinical trials of different VDDs conducted so far have reported low anticancer efficiency at safe plasma levels of the compounds [
7,
8,
11]. Furthermore, since some AML subtypes showed resistance to VDDs in
ex vivo studies, only those patients who are likely to respond would probably benefit from VDD-based differentiation therapy [
7]. A possible way of managing VDD toxicity is combining these compounds at tolerated doses with other agents that would potentiate their anticancer effect but not the calcemic activity.
We and others have shown that different plant antioxidants, such as carotenoids [
12,
13] and polyphenols, e.g., carnosic acid (CA), silibinin and curcumin [
12,
14,
15,
16,
17,
18,
19], can synergistically enhance the differentiation-inducing and antiproliferative effects of various VDDs applied at low (sub)nanomolar concentrations on human and murine AML cell lines and patient-derived AML blasts. Notably, combined treatment with CA-rich rosemary extract and low-calcemic VDDs resulted in cooperative antileukemic effects in syngeneic mouse models of AML without inducing hypercalcemia [
16,
20]. The VDD/CA-induced differentiation was associated with lowered intracellular levels of reactive oxygen species (ROS), upregulated expression of antioxidant enzymes, such as NAD(P)H quinone oxidoreductase-1 (NQO1) and the rate-limiting glutathione-synthesizing enzyme γ-glutamylcysteine synthetase (γGCS), and increased total glutathione content in AML cells [
20,
21,
22]. On the other hand, depletion of cellular glutathione reduced the extent of differentiation [
21].
These findings suggested the role of redox-related mechanisms in the differentiation-enhancing effects of polyphenols. Indeed, we have shown that the CA enhancement is mediated by activation of the Nuclear factor erythroid 2-related factor 2 (Nrf2) transcription factor [
22], a major regulator of the cytoprotective response to electrophilic agents and oxidative stress [
23,
24]. This was demonstrated by manipulating Nrf2 activity and expression in U937 human AML cells stably expressing a dominant-negative Nrf2 mutant (dnNrf2) lacking the transactivation domain and those overexpressing the wild-type Nrf2 [
22]. Further, we found that besides CA, other structurally distinct Nrf2 activators, including the multiple sclerosis drugs dimethyl fumarate (DMF) and monomethyl fumarate (MMF) [
25], synergistically potentiated the antileukemic effects of several VDDs on different AML cell types [
26]. Notably, combined treatment with DMF and the highly potent low-calcemic vitamin D
2 analog PRI-5202 cooperatively inhibited leukemia progression in a xenograft model of AML [
26].
The cooperation between VDDs and Nrf2 activators was associated with a mutual upregulation of VDR, RXRα, and Nrf2 protein levels and activation of VDR and Nrf2 signaling [
22,
26]. Other transcriptional pathways are likely to contribute to this synergy. For instance, we have demonstrated a cooperative upregulation of several Activator Protein 1 (AP-1) family members and augmented DNA binding and transcriptional activity of AP-1 [
15,
22,
27]. A marked upregulation of the Early Growth Response protein 1 (EGR-1) transcription factor was also observed [
15,
27]. Stable expression of the wild-type Nrf2 or dnNrf2 in U937 cells resulted in an enhanced or reduced AP-1 upregulation and activation, respectively [
22], suggesting that Nrf2 may serve as an upstream regulator of AP-1 in AML cells.
The present study was designed to investigate whether glutathione, the most abundant cellular antioxidant and the product of the Nrf2/antioxidant response element (Nrf2/ARE) signaling pathway [
24], may mediate the enhancing effects of Nrf2 activators on VDD-induced differentiation of AML cells. To this end, we employed two approaches: 1) glutathione depletion in nontransfected HL60 cells using BSO and 2) repletion of reduced glutathione (GSH) levels in dnNrf2-expressing HL60 cells by adding membrane-permeant GSH ethyl ester (GEE). We found that co-treatment with BSO attenuated the potentiating effect of both CA and MMF on 1,25D
3-induced differentiation. This was paralleled by the downregulation of VDR-responsive genes, the AP-1 family protein c-Jun, and its phosphorylation. On the other hand, the addition of GEE partially reversed the suppressing effects of dnNrf2 on cell differentiation, vitamin D- and Nrf2-related gene and/or protein expression, and c-Jun and P-c-Jun levels. Finally, using the transcription factor decoy strategy [
22,
28], we demonstrated that cell loading with AP-1-specific oligodeoxynucleotide (ODN) markedly inhibited the enhancing effects of CA and MMF on 1,25D
3-induced expression of myeloid differentiation markers and VDR and RXRα proteins, and transactivation of the vitamin D response element (VDRE).
3. Discussion
Accumulating evidence indicates that the transcription factors Nrf2 [
32,
33,
34,
35,
36] and AP-1 [
28,
37,
38,
39,
40,
41,
42] play significant roles in the differentiation of various normal and cancer cell types, including hematopoietic cells. We have previously reported that the antileukemic synergy between VDDs and Nrf2 activators is associated with a mutual upregulation of VDR and Nrf2 signaling [
22,
26] and that Nrf2 may function as an upstream regulator of VDR, RXRα and AP-1 protein levels in AML cells [
22]. However, the mode of the interaction between the Nrf2, AP-1 and VDR/RXRα pathways remains unclear. The present study was designed to explore the role of glutathione as the potential mediator of the differentiation-enhancing effects of Nrf2 activators in this system.
Glutathione is a ubiquitous thiol tripeptide formed by glutamic acid, cysteine, and glycine. It is synthesized in the cytosol by consecutive action of two enzymes, γ-GCS (the rate-limiting enzyme) and glutathione synthetase, and reaches millimolar intracellular concentrations [
24,
31]. Nrf2, AP-1, and nuclear factor kappa B (NFκB) are among the key transcription factors that regulate the expression of these enzymes [
31]. The reduced form of glutathione (GSH) functions as the principal cellular reducing agent and antioxidant and participates in various regulatory processes, including cytoprotection, cell signaling, metabolism of xenobiotics, gene expression, protein synthesis and modification, cell cycle, apoptosis, and immunomodulation [
24,
43,
44,
45,
46].
Glutathione depletion has been shown to impair the differentiation of various cell types. For instance, Esposito et al. [
47] reported that the glutathione-conjugating compound diethyl maleate inhibited 12-O-tetradecanoylphorbol-13-acetate (TPA)-driven differentiation of HL60 and KG-1 AML cells. We showed that the specific γGCS inhibitor BSO attenuates 1,25D
3- and 1,25D
3/CA-induced differentiation of HL60 cells [
21]. Similar results were obtained in other cell types, such as T cells [
48], dendritic cells [
49], macrophages [
50], C2C12 skeletal muscle cells [
51], osteoblasts [
52], and osteoclasts [
53]. These data indicate an essential role of glutathione in cell maturation.
The main findings of the present study are summarized below and schematically represented in
Figure 9.
3.1. Involvement of glutathione in the regulation of VDR signaling and myeloid differentiation
We found that glutathione depletion and repletion had opposite impacts on the induction of monocytic differentiation of HL60 cells. Although BSO minimally attenuated the 1,25D3-induced surface expression of CD14 and CD11b, it significantly suppressed the enhancing effects of both Nrf2 activators. This correlated with decreased induction of CD14 and ITGAM and two other known vitamin D-responsive genes, CAMP and CYP24A1. Notably, target gene expression was inhibited without noticeably reducing mRNA and protein levels of VDR and RXRα, suggesting that glutathione depletion primarily inhibited VDR transcriptional activity in our system.
In contrast to BSO, exogenous GSH (as GEE) or, to a lesser extent, its precursor NAC augmented cell differentiation induced by paricalcitol and its combination with CA. This effect was especially pronounced in dnNrf2-expressing HL60 cells, which exhibited a relatively lower level of differentiation and glutathione content than vector-transfected cells. The boosted differentiation was accompanied by the increased expression of both VDR protein and all the vitamin D target genes tested. The above results indicate that glutathione positively regulates VDR transcriptional activity and can mediate, at least in part, the enhancing effect of Nrf2 activators on VDD-induced differentiation of HL60 cells.
Interestingly, using similar approaches, Fujita et al. [
53] have shown that BSO suppresses TNFα-stimulated osteoclast differentiation
in vitro, while exogenous GSH promotes it both in cell culture and a mouse model of lipopolysaccharide-induced osteoclastogenesis. These opposite effects were associated with corresponding changes in the nuclear localization of the Nuclear Factor of Activated T cells c1 (NFATc1), a master regulator of osteoclastogenesis, and the expression of osteoclast-specific genes [
53].
3.2. Modulation of glutathione levels and Nrf2/ARE signaling: Role of the intracellular ROS accumulation
Cellular Nrf2 levels are primarily regulated by Kelch-like ECH-associated protein 1 (Keap-1), a subunit of Cullin 3 (CUL3)-based E3 ubiquitin ligase. Under physiological conditions, Keap-1 physically binds Nrf2 and promotes its proteasomal degradation. ROS and electrophilic compounds, e.g., the quinone form of CA [
54] and fumaric acid esters, react with cysteine SH-groups of Keap-1, triggering dissociation and cellular accumulation of Nrf2 [
23,
55,
56].
Accordingly, treating intact or vector-transfected HL60 cells with CA or MMF increased Nrf2 protein levels and the expression of Nrf2 target genes and encoded proteins, including γGCS subunits. This correlated with an increase in the total glutathione levels. As expected, both dnNrf2-expressing and BSO-treated cells had lower basal and induced glutathione levels than the corresponding reference cells. However, only in dnNrf2-HL60 cells was this associated with impaired induction of Nrf2 and its target gene products. In contrast, BSO-treated cells exhibited enhanced induction of most tested genes and encoded proteins attributed to the Nrf2/ARE pathway. The latter could be due to the compensatory upregulation of this and other redox-sensitive regulatory pathways in response to ROS accumulation caused by pharmacological inhibition of γGCS enzymatic activity.
Unexpectedly, GEE was also found to act as a prooxidant, but only in dnNrf2-HL60 cells with impaired antioxidant defense, even though we used the compound at a much lower concentration (0.25 mM) compared to other studies (1.0-5.0 mM) [
57,
58,
59,
60]. This effect might be due to reductive stress, which can result in excess ROS generation [
61,
62,
63]. Nonetheless, both dnNrf2-HL60 cells and pEF-HL60 cells exhibited enhanced induction of Nrf2-related genes and proteins when co-treated with GEE. Therefore, GSH appears to promote Nrf2/ARE activation by electrophilic agents independently of the cytosolic ROS levels, but ROS accumulation in GEE-treated dnNrf2-HL60 cells may have an additional positive effect on this pathway.
3.3. Modulation of c-Jun by glutathione and the role of AP-1 in differentiation enhancement
The transcription factor AP-1 is a dimeric protein complex composed of transcription factors belonging to the Jun, Fos, ATF, and Maf families, which controls the expression of various genes regulating cell proliferation, cell cycle, apoptosis, and differentiation [
37,
64,
65]. It has also been established that AP-1 can be upregulated and activated by ROS to induce the expression of antioxidant and detoxifying enzymes [
66,
67]. Furthermore, c-Jun binding to the
NFE2L2 promoter was found to transcriptionally upregulate Nrf2, leading to an antioxidant effect [
68]. Additionally, c-Jun can dimerize with Nrf2 and activate Nrf2/ARE-induced transcription [
69].
Here, we demonstrated that, consistent with our previous findings [
22,
27], VDDs and CA strongly cooperated in increasing c-Jun protein expression and phosphorylation. On the other hand, MMF was quite active alone, particularly in elevating P-c-Jun levels. Interestingly, another fumaric acid ester, DMF, was shown to differentially affect c-Jun in a cell type- and treatment-dependent manner. For instance, DMF upregulated c-Jun and P-c-Jun levels in macrophage migration inhibitory factor-stimulated human keratinocytes [
70] but inhibited hypoxia-induced c-Jun phosphorylation in endothelial cells [
71].
It was previously reported that inhibition of TPA-induced differentiation of AML cells by glutathione depletion was associated with a reversible reduction in DNA binding of AP-1 [
47]. In line with these data, we found that both BSO treatment and dnNrf2 expression reduced c-Jun and P-c-Jun levels in our experimental system. Although both BSO and GEE induced ROS generation and promoted Nrf2 signaling in HL60 cells, glutathione depletion and repletion had opposite effects on c-Jun levels and phosphorylation. These results suggest that it is glutathione, and probably not other Nrf2/ARE activities, that positively regulates AP-1. By exploiting the transcription factor decoy strategy [
22,
28,
72], we obtained evidence supporting the mediatory function of AP-1 in the differentiation-enhancing effects of the Nrf2 activators, probably
via upregulating VDR/RXRα levels and transcriptional activity.
3.4. A possible role of proteasome inhibition in a cooperative upregulation of VDR and Nrf2 protein expression by VDDs and Nrf2 activators
It has been reported that in 1,25D
3-treated HL60 cells, VDR protein levels are elevated without significant changes in
VDR gene expression [
73,
74]. Here, we observed a similar lack of
VDR induction by VDDs alone and also when VDR protein levels were synergistically increased by adding Nrf2 activators. These data indicate that VDR upregulation occurred at posttranscriptional or posttranslational levels. It was previously suggested that liganded VDR undergoes conformational changes, which prevent its proteolysis [
75,
76]. Indeed, several studies have demonstrated that 1,25D
3 upregulates VDR by protecting it from proteasomal degradation [
77,
78,
79]. Interestingly, 1,25D
3 was also found to promote Nrf2 accumulation in bone marrow mesenchymal stem cells by inhibiting its degradation
via transcriptionally repressing Keap-1 [
80].
Various natural polyphenolic compounds have been shown to inhibit or activate the ubiquitin/proteasome pathway depending on multiple factors [
81]. For instance, resveratrol acted as a proteasome inhibitor in breast cancer cells, inducing the accumulation of the ∆16HER2 splice variant of HER-2 [
82], but promoted proteasomal degradation of Nanog in glioma stem cells [
83]. These effects are also dose-dependent, e.g., curcumin increases proteasome activity at low concentrations (≤1 µM) but inhibits it at high concentrations (≥10 µM) [
84].
Although both CA and MMF can activate the Nrf2/ARE pathway by inducing Keap-1-Nrf2 dissociation, which results in Nrf2 protein stabilization, CA has been shown to promote proteasomal degradation of other proteins in cancer cells (e.g., [
85,
86]). The major oxidized CA metabolite, carnosol, was found to target several proteins to proteasome degradation in breast cancer cells [
87,
88] and to directly inhibit proteasome activity in colon cancer cells [
89]. DMF was shown to promote protein degradation in fibroblasts [
71,
90]. However, both DMF and MMF enhanced the cytotoxic effect of proteasome inhibitors in other cell types [
91,
92].
To the best of our knowledge, there have been no reports on regulating VDR protein proteolysis by polyphenols and fumaric acid esters. Still, CA (or its oxidized metabolites) and MMF could potentially inhibit VDR proteasomal degradation in VDD-treated HL60 cells, which enhanced VDR protein, but not mRNA, expression following combined treatments. Conversely, VDDs might cooperate with the electrophilic compounds to stabilize Nrf2. In addition, our data demonstrate that treatment with exogenous GSH alone upregulated most proteins tested in this study, suggesting a contribution of a general activation of protein synthesis or stabilization under these conditions.
4. Materials and Methods
4.1. Materials
Carnosic acid (>98%) was obtained from ShenZhen Ipure Biological Import and Export Co., Ltd. (Shenzhen, China). 1,25D3 was purchased from Selleck Chemicals (Houston, TX). Monomethyl fumarate (MMF) and DMSO were obtained from Sigma Chemical Co. (St. Louis, MO). Paricalcitol and glutathione ethyl ester (GEE) were from Cayman Chemical (Ann Arbor, MI, USA). The antibodies against VDR (D-6 and C-20), RXRα (D-20), NQO-1 (A-5), ɣ-GCSc (H-5), ɣ-GCSm (E-4), TrxR1 (B-2), and 2’,7’-dichlorofluorescein-diacetate (DCFH-DA) were procured from Santa Cruz Biotechnology Inc. (Dallas, TX). Antibodies against P-c-Jun (54B3), c-Jun (60AB), and HO-1 (D60611) were acquired from Cell Signaling Technology (Danvers, MA, USA). Antibodies against Nrf2 (MAB3925) and calreticulin (PA3-900) were purchased from R&D Systems (Minneapolis, MN, USA) and Thermo Fisher Scientific (Waltham, MA, USA), respectively. Peroxidase-conjugated AffiniPure donkey anti-rabbit and sheep anti-mouse IgG antibodies were bought from Jackson ImmunoResearch Laboratories, Inc. (West Grove, PA). L-buthionine-sulfoximine (BSO) was obtained from Merck-Sigma-Aldrich (Rehovot, Israel). Hanks’ buffered salt solution (HBSS), penicillin, streptomycin, and HEPES buffer were from IMBH (Beth Haemek, Israel). RPMI 1640 medium and heat-inactivated fetal bovine serum (FBS) were purchased from Gibco-Invitrogen (Carlsbad, CA, USA). Stock solutions of 1,25D3 and paricalcitol (2.4 mM), CA (10 mM), and MMF (50 mM) were prepared in absolute ethanol. The precise concentration of 1,25D3 in ethanol was verified spectrophotometrically at 264 nm (ε=19,000).
4.2. Cell culture and stable transfection
HL60 myeloblastic leukemia cells (ATCC-CCL-240) were grown in RPMI 1640 medium supplemented with 10% fetal calf serum (FCS), penicillin (100 U/ml), streptomycin (0.1 mg/ml), and 10 mM HEPES (pH = 7.4) in a humidified atmosphere of 95% air and 5% CO
2 at 37°C. The expression vector for dnNrf2, which lacks the transactivation domain residues 1-392 in the NH
2-terminal portion of the protein, and the empty vector (pEF) were generously provided by Dr. Jawed Alam (Louisiana State University Medical Center, New Orleans, LA). Both plasmids carried the neomycin (
neoR) resistance gene. Stable nucleofection was performed by Dr. Irene Bobilev (Ben Gurion University of the Negev), as described previously [
22]. Briefly, HL60 cells (1 × 10
7 cells/ml) were mixed with 1 µg plasmid in Cell Line Nucleofector Solution V and transfected in an Amaxa Nucleofector (Lonza, Cologne, Germany) according to the manufacturer’s protocol (program T-19).
4.3. Determination of cell differentiation markers
Cells were seeded at 1 × 10
5 cells/ml and treated with test agents or vehicle (<0.2% ethanol) for 48 h. Cell numbers and viability were determined using the trypan blue exclusion assay by enumeration in a Vi-Cell XR cell viability analyzer (Beckman Coulter Inc., Fullerton, CA, USA). Aliquots of 5 × 10
5 cells were harvested, washed with PBS, and incubated for 45 min at room temperature with 0.3 µl MO1-FITC and 0.3 µl MY4-RD1 (Beckman Coulter) to determine the expression of myeloid surface antigens CD11b and CD14, respectively, by flow cytometry as described previously [
18,
21]. For each analysis, 10,000 events were recorded, and the data were processed using Kaluza software, version 2.1 (Beckman Coulter).
4.4. Determination of intracellular levels of reactive oxygen species
Cytosolic ROS levels were determined using the oxidation-sensitive fluorescent probe DCFH-DA. Intracellular ROS oxidize this probe to a highly fluorescent compound, DCF. Following treatments with the specified compounds at the indicated time points, cells were washed with HBSS containing 10 mM HEPES buffer (pH=7.4). Subsequently, cells were stained with 5 μM DCFH-DA for 15 min at 37°C in the dark using a shaking water bath and washed with HEPES-buffered HBSS. For the positive control, DCFH-DA-loaded cells were treated with 0.5 mM H
2O
2 for 15 min. Untreated and unstained cells served as the negative control. In the experiment reported in
Figure 5(c,d), DCFH-DA-loaded cells were divided into two groups and incubated with vehicle (HBSS) or 10 µM of H
2O
2 for 15 min, followed by washing with HEPES-buffered HBSS. The DCF fluorescence intensity was measured by flow cytometry, recording 10,000 events for each analysis. Data were analyzed using Kaluza Analysis Software version 2.1 (Beckman Coulter) [
57].
4.5. Preparation of whole cell lysates and western blotting
Cells were seeded at 1 × 10
5 cells/ml and incubated with test agents or vehicle (<0.2% ethanol) for 48 h. Preparation of whole cell lysates and Western blotting analysis were performed as described previously [
18]. Briefly, cells were lysed in a buffer containing 1% (v/v) Triton X-100 at 4°C, subjected to SDS-PAGE, and electroblotted into nitrocellulose membranes. The membranes were exposed to primary antibodies overnight at 4°C. Blots were washed and incubated with horse-radish peroxidase-conjugated secondary antibodies. Membranes were stripped and reprobed for calreticulin, the internal loading control. The protein bands were visualized using the WESTAR ANTARES Chemiluminescent Substrate for Western blotting (Cyanagen, Bologna, Italy). The absorbance of each band was determined using the Image Quant LAS 4000 system (GE Healthcare, Little Chalfont, UK).
4.6. RNA extraction, cDNA synthesis, and RT-qPCR
Total RNA was purified from cell cultures according to the manufacturer’s instructions using an RNA extraction kit (GENEzolTM TriRNA Pure Kit+DNASE I; Geneaid, New Taipei City, Taiwan). A micro-volume spectrophotometer (NanoDrop; Wilmington, DE, USA) was used for RNA quantification. First-strand cDNA was generated by reverse transcriptase kit (qScript cDNA synthesis kit; QUANTA Biosciences; Gaithersburg, MD, USA) using random oligo (dT) after a sample concentration was normalized. Quantitative cDNA amplification was performed by real-time PCR (StepOne Real-Time PCR System; Thermo Fisher Scientific; Wilmington, DE, USA) using qPCRBIO Fast qPCR SyGreen Blue Mix from Tamar Laboratory Supplies Ltd (Mevaseret Zion, Israel). Relative mRNA expression levels were determined using the 2-(ΔΔCt) formula, where ΔCt is Ct (target gene) - mean of Ct (reference genes). The reference gene used in this study was GAPDH. Each experiment was performed using three biological replicates, each assayed in triplicate. Primers for qPCR were synthesized by Hy Laboratories Ltd. (Rehovot, Israel) and Tamar Laboratory Supplies Ltd. (Mevaseret Zion, Israel).
The primer sequences used in this study were as follows:
CAMP FR |
GCTAACCTCTACCGCCTCCT |
CAMP REV |
GGTCACTGTCCCCATACACC |
CD14 FR |
CAACCTAGAGCCGTTTCTAAAGC |
CD14 REV |
GCGCCTACCAGTAGCTGAG |
CYP24A1 FR |
GGAAGTGATGAAGCTGGACAACA |
CYP24A1 REV |
CTCATACAACACGAGGCAGATAC |
GAPDH FR |
CATGAGAAGTATGACAACAGCCT |
GAPDH REV |
AGTCCTTCCACGATACCAAAGT |
GCLC FR |
GGAGGAAACCAAGCGCCAT |
GCLC REV |
CTTGACGGCGTGGTAGATGT |
GCLM FR |
GGAAGAAGTGCCCGTCCA |
GCLM REV |
CTGAACAGGCCATGTCAACT |
HO-1 FR |
AAGACTGCGTTCCTGCTCAA |
HO-1 REV |
GGTCCTTGGTGTCATGGGTC |
ITGAM FR |
CTGTCTGCCAGAGAATCCAGTG |
ITGAM REV |
GAGGTGGTTATGCGAGGTCTTG |
NQO1 FR |
AAAGAAGGCCATCTGAGCCC |
NQO1 REV |
CCAGGCGTTTCTTCCATCCT |
Nrf2 FR |
CCTTGTCACCATCTCAGGGG |
Nrf2 REV |
TGGGGTTTTCCGATGACCAG |
TXNRD1 FR |
ACGTTACTTGGGCATCCCTG |
TXNRD1 REV |
AGAAATCCAGCGCACTCCAA |
VDR FR |
GACCTGTGGCAACCAAGACT |
VDR REV |
AATCAGCTCCAGGCTGTGTC |
4.7. Total glutathione assay
Cells (2 × 10
6) were collected by centrifugation (1,000×g for 5 min), washed with ice-cold PBS, and resuspended in 200 µl of 5% 5-sulfosalicylic acid. After 15 min on ice with intermittent vortexing, the suspension was centrifuged at 16,000×g for 5 min to remove protein precipitates. Total glutathione was determined in the supernatants by the glutathione reductase recycling assay [
21,
22]. The rates of 5-thio-2-nitrobenzoic acid (TNB) formation were measured kinetically at 412 nm for 30 min using VersaMax microplate spectrophotometer (Molecular Devices).
4.8. Cell treatment with AP-1 decoy oligodeoxynucleotides
HL60 cells were preincubated for 24 h with 10 µM double-stranded phosphorothioate oligodeoxynucleotide containing the proximal binding site for AP-1 (TPA-response element; TRE) from the promoter region of hVDR (-77 to -97 relative to the transcription start site; 5′- CTG GCA AGA GAG
GAC TGG ACC-3′) or its mutant (5′-CTG GCA AGA GAG
TGC TGG ACC-3′) in the complete culture medium, followed by treatment with test agents for an additional 48 h [
22]. The oligonucleotides were synthesized by Integrated DNA Technologies, lnc. (Coralville, IA).
4.9. Transient transfection and reporter gene assay
Cells were harvested after 48 h of culture, washed once in a preheated growth medium at 37°C, and resuspended at 5 × 10
5 cells/ml. Four hundred μl of the cell suspension were transferred to 24-well plates, followed by co-transfection with 0.8 µg VDREx6-Luc luciferase reporter plasmid and 0.2 µg
Renilla luciferase (pRL-null) reporter plasmid (internal control) using jetPEI reagent (Polyplus Transfection, Illkrich, France). Four hours later, cells were pre-incubated for 24 h with either a vehicle, 10 µM TRE-ODN, or mutant TRE (mTRE)-ODN. Subsequently, they were treated for an additional 48 h with 1 nM 1,25D
3, 10 µM CA or 50 µM MMF, alone or in combination. Firefly and
Renilla luciferase activities were then measured using the Dual-Luciferase Reporter Assay system (Promega, Madison, WI, USA) in accordance with the manufacturer’s instructions. Luminescence was determined using a Turner 20/20 luminometer (Turner BioSystems, Sunnyvale, CA, USA). Data are expressed as firefly luciferase-to-
Renilla luciferase ratios (RLU) [
22]. The VDREx6-Luc reporter plasmid was gifted by Dr. David G. Garner (University of California, San Francisco, CA). The pRL-null vector was purchased from Promega (Madison, WI, USA).
4.10. Statistical analysis
All experiments were performed at least three times. Statistically significant differences between the two experimental groups were assessed using one-way ANOVA. Data are presented as the mean ± SD. p < 0.05 was considered statistically significant. The synergy between the effects of two compounds (A and B) was assumed if the effect of their combination (AB) was larger than the sum of their individual effects (AB>A+B), the data being compared after subtraction of the respective control values from A, B, and AB [
15]. The statistical analyses were performed using GraphPad Prism 6.0 software (Graph-Pad Software, San Diego, CA, USA).
Figure 1.
Glutathione depletion by buthionine sulfoximine inhibits the potentiating effects of Nrf2 activators on 1,25D3-induced differentiation and elevates ROS levels in HL60 cells. Cells were preincubated with vehicle (water) or 30 μM BSO for 24 h, followed by treatment with the indicated concentrations of 1,25D3, carnosic acid (CA), or monomethyl fumarate (MMF), alone or in combination, for another 48 h. (a) Representative flow cytometric data showing the enhancing effect of CA on 1,25D3-induced surface expression of CD14 and CD11b and the inhibitory effect of BSO on this enhancement. (b) Summarized CD14 and CD11b expression data, as exemplified in panel a. (c) Changes in the total glutathione content, as determined by the glutathione reductase recycling assay following 24 h of preincubation with BSO and 16 h of treatment with CA or MMF. (d) Averaged ROS levels measured as DCF geometric mean fluorescence intensity (MFI) units (% of control). Cells were preincubated with BSO for 24 h and treated with CA or MMF for 48 h. The data are means ± SD of at least 3 independent experiments performed in duplicate. *, p < 0.05; **, p < 0.01; ****, p < 0.0001 vs. untreated control group; ##, p < 0.01; ###, p < 0.001 vs. sum of the effects of single agents; $$, p < 0.01; $$$, p < 0.001; $$$$, p < 0.0001 vs. corresponding BSO-untreated group.
Figure 1.
Glutathione depletion by buthionine sulfoximine inhibits the potentiating effects of Nrf2 activators on 1,25D3-induced differentiation and elevates ROS levels in HL60 cells. Cells were preincubated with vehicle (water) or 30 μM BSO for 24 h, followed by treatment with the indicated concentrations of 1,25D3, carnosic acid (CA), or monomethyl fumarate (MMF), alone or in combination, for another 48 h. (a) Representative flow cytometric data showing the enhancing effect of CA on 1,25D3-induced surface expression of CD14 and CD11b and the inhibitory effect of BSO on this enhancement. (b) Summarized CD14 and CD11b expression data, as exemplified in panel a. (c) Changes in the total glutathione content, as determined by the glutathione reductase recycling assay following 24 h of preincubation with BSO and 16 h of treatment with CA or MMF. (d) Averaged ROS levels measured as DCF geometric mean fluorescence intensity (MFI) units (% of control). Cells were preincubated with BSO for 24 h and treated with CA or MMF for 48 h. The data are means ± SD of at least 3 independent experiments performed in duplicate. *, p < 0.05; **, p < 0.01; ****, p < 0.0001 vs. untreated control group; ##, p < 0.01; ###, p < 0.001 vs. sum of the effects of single agents; $$, p < 0.01; $$$, p < 0.001; $$$$, p < 0.0001 vs. corresponding BSO-untreated group.
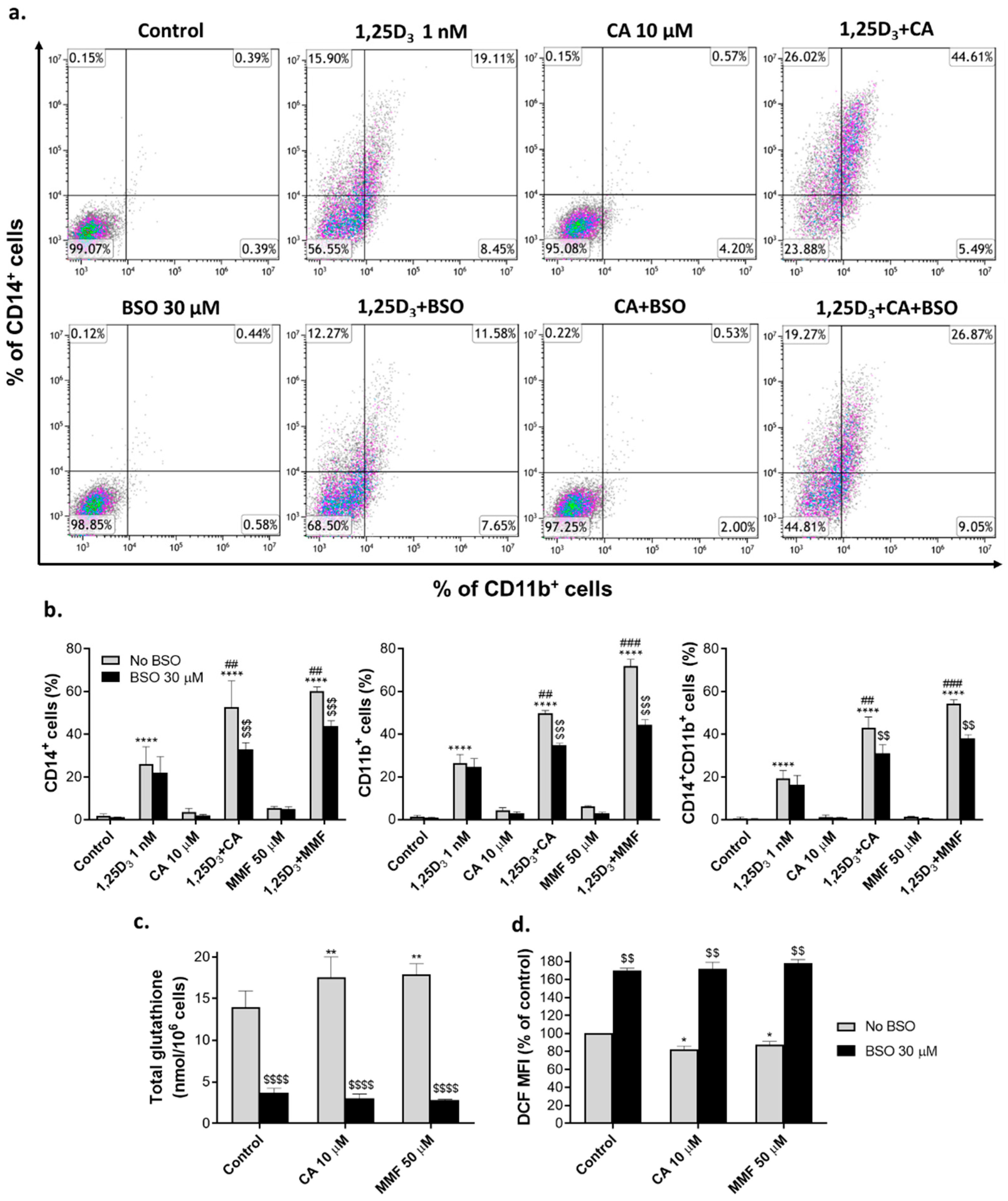
Figure 2.
Effects of glutathione depletion on mRNA levels of vitamin D- and Nrf2-related genes. HL60 cells were incubated with the indicated agents for 48 h, as described in the legend to
Figure 1. Cell samples were then analyzed for mRNA levels of the indicated genes using quantitative RT-PCR. The expression of specific genes was normalized by the C
T value of the internal reference gene (
GAPDH). ). The data are means ± SD of 3 experiments performed in triplicate. *, p < 0.05; **, p < 0.01; ***, p < 0.001; ****, p < 0.0001
vs. untreated control group; #, p < 0.05; ###, p < 0.001; ####, p < 0.0001
vs. sum of the effects of single agents;
$, p < 0.05;
$$, p < 0.01;
$$$, p < 0.001
vs. corresponding BSO-untreated group.
Figure 2.
Effects of glutathione depletion on mRNA levels of vitamin D- and Nrf2-related genes. HL60 cells were incubated with the indicated agents for 48 h, as described in the legend to
Figure 1. Cell samples were then analyzed for mRNA levels of the indicated genes using quantitative RT-PCR. The expression of specific genes was normalized by the C
T value of the internal reference gene (
GAPDH). ). The data are means ± SD of 3 experiments performed in triplicate. *, p < 0.05; **, p < 0.01; ***, p < 0.001; ****, p < 0.0001
vs. untreated control group; #, p < 0.05; ###, p < 0.001; ####, p < 0.0001
vs. sum of the effects of single agents;
$, p < 0.05;
$$, p < 0.01;
$$$, p < 0.001
vs. corresponding BSO-untreated group.
Figure 3.
Effects of glutathione depletion on the levels of vitamin D- and Nrf2-related proteins, c-Jun and its phosphorylation. HL60 cells were incubated with the indicated agents, as described in the legend to
Figure 1. Cell samples were analyzed by Western blotting. Calreticulin was used as the protein loading control. (
a, j) Representative Western blot images.
(b-i, k, l) Absorbance values for specific proteins were normalized to those of calreticulin and expressed in the bar graphs as fold change relative to the corresponding untreated controls. The data are means ± SD of 3 experiments. *, p < 0.05; **, p < 0.01; ***, p < 0.001
vs. untreated control cells; #, p < 0.05; ##, p < 0.01; ###, p < 0.001
vs. sum of the effects of single agents;
$, p < 0.05;
$$, p < 0.01;
$$$, p < 0.001
vs. corresponding BSO-untreated group.
Figure 3.
Effects of glutathione depletion on the levels of vitamin D- and Nrf2-related proteins, c-Jun and its phosphorylation. HL60 cells were incubated with the indicated agents, as described in the legend to
Figure 1. Cell samples were analyzed by Western blotting. Calreticulin was used as the protein loading control. (
a, j) Representative Western blot images.
(b-i, k, l) Absorbance values for specific proteins were normalized to those of calreticulin and expressed in the bar graphs as fold change relative to the corresponding untreated controls. The data are means ± SD of 3 experiments. *, p < 0.05; **, p < 0.01; ***, p < 0.001
vs. untreated control cells; #, p < 0.05; ##, p < 0.01; ###, p < 0.001
vs. sum of the effects of single agents;
$, p < 0.05;
$$, p < 0.01;
$$$, p < 0.001
vs. corresponding BSO-untreated group.
Figure 4.
Stable expression of dominant-negative Nrf2 suppresses the differentiation induced by paricalcitol and its combination with carnosic acid and reduces glutathione levels. Cells stably transfected with either empty vector (pEF-HL60) or dominant-negative Nrf2 (dnNrf2-HL60) were incubated with the indicated concentrations of paricalcitol (19-D2) and carnosic acid (CA), alone or combination, for 48 h. (a) Representative flow cytometric data showing changes in CD14 and CD11b surface expression. (b) Summarized CD14 and CD11b expression data exemplified in panel a. (c) pEF-HL60 and dnNrf2-HL60 cells differ in the total glutathione content. Cells were treated with vehicle or the indicated concentrations of CA for 48 h. The total cellular glutathione (GSH+GSSG) concentration was determined by the glutathione reductase recycling assay. The data are means ± SD of at least 3 independent experiments performed in duplicate. *, p < 0.05; **, p < 0.01; ***, p < 0.001; ****, p < 0.0001 vs. corresponding control group; ##, p < 0.01; ###, p < 0.001 vs. corresponding sum of the effects of single agents; ^, < p < 0.05; ^^, <p < 0.01, dnNrf2-HL60 cells vs. pEF-HL60 cells.
Figure 4.
Stable expression of dominant-negative Nrf2 suppresses the differentiation induced by paricalcitol and its combination with carnosic acid and reduces glutathione levels. Cells stably transfected with either empty vector (pEF-HL60) or dominant-negative Nrf2 (dnNrf2-HL60) were incubated with the indicated concentrations of paricalcitol (19-D2) and carnosic acid (CA), alone or combination, for 48 h. (a) Representative flow cytometric data showing changes in CD14 and CD11b surface expression. (b) Summarized CD14 and CD11b expression data exemplified in panel a. (c) pEF-HL60 and dnNrf2-HL60 cells differ in the total glutathione content. Cells were treated with vehicle or the indicated concentrations of CA for 48 h. The total cellular glutathione (GSH+GSSG) concentration was determined by the glutathione reductase recycling assay. The data are means ± SD of at least 3 independent experiments performed in duplicate. *, p < 0.05; **, p < 0.01; ***, p < 0.001; ****, p < 0.0001 vs. corresponding control group; ##, p < 0.01; ###, p < 0.001 vs. corresponding sum of the effects of single agents; ^, < p < 0.05; ^^, <p < 0.01, dnNrf2-HL60 cells vs. pEF-HL60 cells.
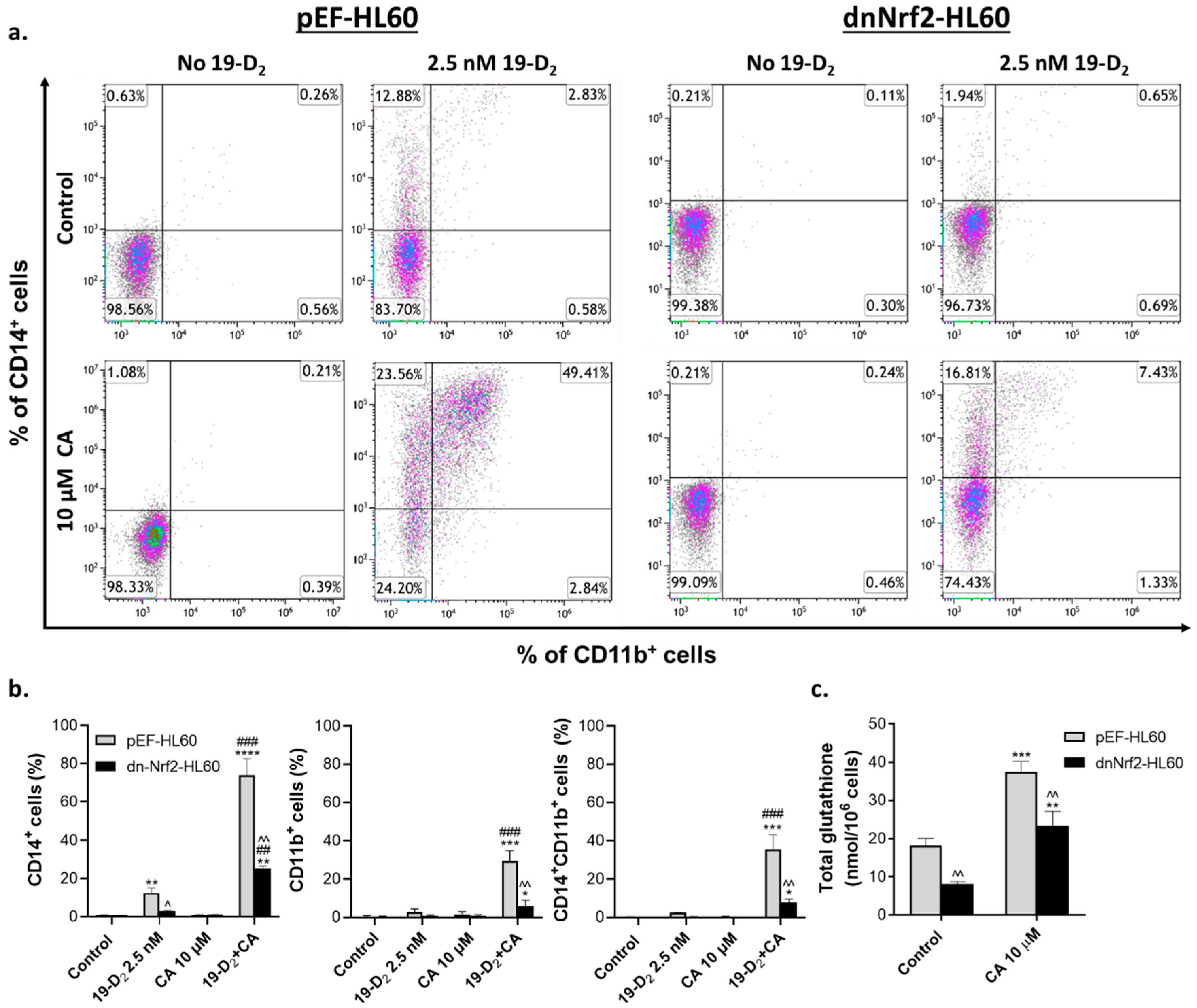
Figure 5.
GSH ethyl ester, but not Trolox, reverses the inhibitory effect of dominant-negative Nrf2 on the differentiation of HL60 cells while increasing cytosolic ROS levels. (a, b) Effects of GEE or Trolox on cell differentiation. Cells were pre-incubated with vehicle, GEE or Trolox for 1 h, followed by adding paricalcitol, CA or their combination and incubating for another 48 h. The expression of CD14 and CD11b was determined by flow cytometry. *, p < 0.05; **, p < 0.01; ***, p < 0.001; ****, p < 0.0001, vs. corresponding control group; #, p < 0.05; ##, p < 0.01; ###, p < 0.001, vs. corresponding sum of the effects of single agents; $, p < 0.05; $$, p < 0.01, GEE-treated vs. corresponding GEE-untreated group. (c, d) Changes in cytosolic ROS levels. Cells were incubated with vehicle, GEE or Trolox for 48 h, followed by exposure to 10 µM H2O2 for an additional 15 min. The results are expressed as DCF geometric mean fluorescence intensity (MFI) units (% of control). The data are means ± SD of 3 independent experiments. **, p < 0.01; ***, p < 0.001; ****, p < 0.0001, vs. corresponding H2O2-untreated group; $, p < 0.05; $$, p < 0.01; $$$, p < 0.001 vs. corresponding GEE- or Trolox-untreated group.
Figure 5.
GSH ethyl ester, but not Trolox, reverses the inhibitory effect of dominant-negative Nrf2 on the differentiation of HL60 cells while increasing cytosolic ROS levels. (a, b) Effects of GEE or Trolox on cell differentiation. Cells were pre-incubated with vehicle, GEE or Trolox for 1 h, followed by adding paricalcitol, CA or their combination and incubating for another 48 h. The expression of CD14 and CD11b was determined by flow cytometry. *, p < 0.05; **, p < 0.01; ***, p < 0.001; ****, p < 0.0001, vs. corresponding control group; #, p < 0.05; ##, p < 0.01; ###, p < 0.001, vs. corresponding sum of the effects of single agents; $, p < 0.05; $$, p < 0.01, GEE-treated vs. corresponding GEE-untreated group. (c, d) Changes in cytosolic ROS levels. Cells were incubated with vehicle, GEE or Trolox for 48 h, followed by exposure to 10 µM H2O2 for an additional 15 min. The results are expressed as DCF geometric mean fluorescence intensity (MFI) units (% of control). The data are means ± SD of 3 independent experiments. **, p < 0.01; ***, p < 0.001; ****, p < 0.0001, vs. corresponding H2O2-untreated group; $, p < 0.05; $$, p < 0.01; $$$, p < 0.001 vs. corresponding GEE- or Trolox-untreated group.
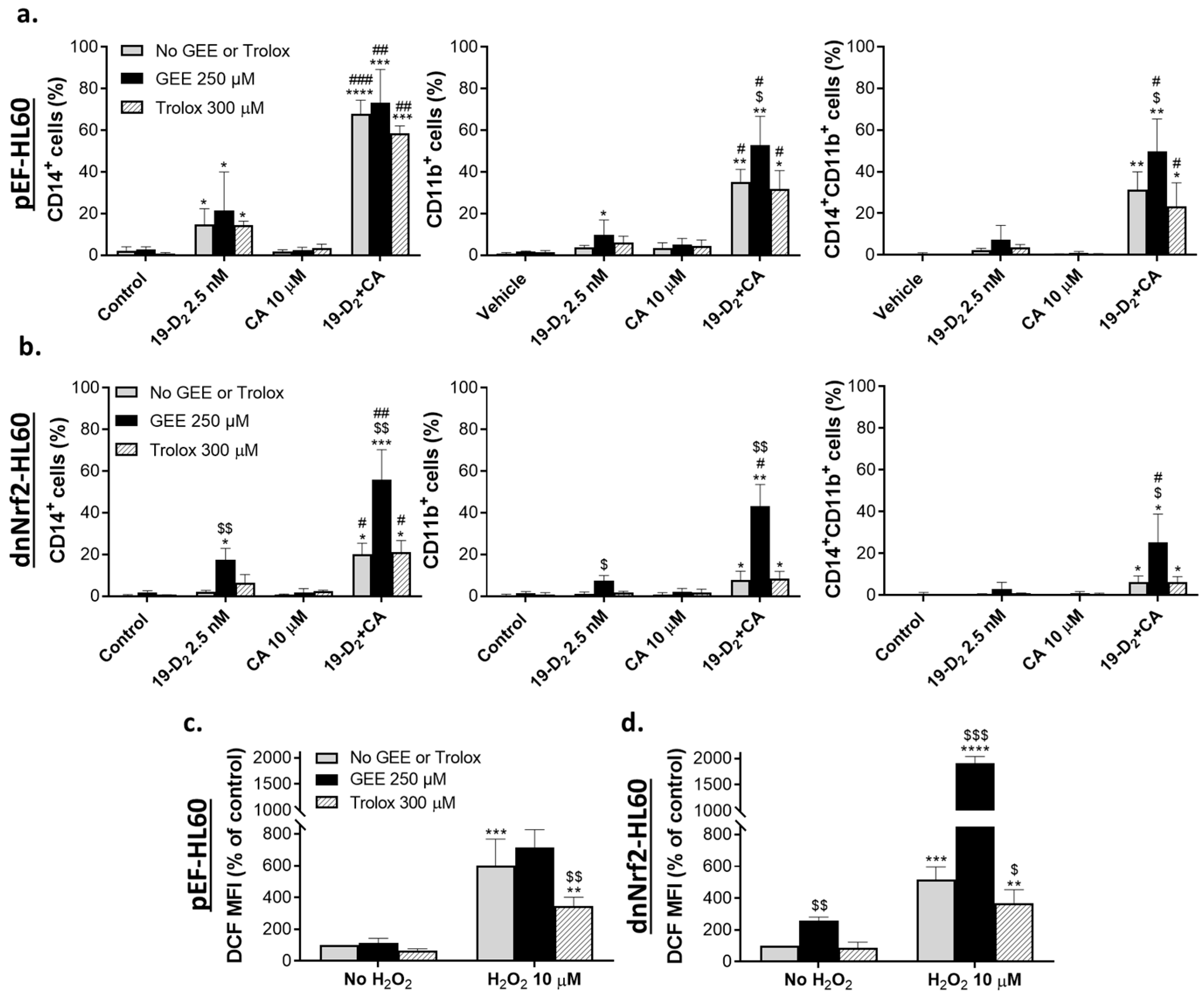
Figure 6.
Effects of GSH ethyl ester on mRNA levels of vitamin D- and Nrf2-related genes. pEF-HL60 and dnNrf2-HL60 cells were incubated with the indicated agents for 48 h. Samples were analyzed for mRNA levels of the indicated genes using quantitative RT-PCR. The expression of specific genes is normalized by the CT value of the internal reference gene (GAPDH). The data are means ± SD of 3 experiments performed in triplicate. *, p < 0.05; **, p < 0.01; ***, p < 0.001; ****, p < 0.0001 vs. corresponding untreated control group; #, p < 0.05; ##, p < 0.01; ###, p < 0.001; ####, p < 0.0001 vs. corresponding sum of the effects of single agents. $, p < 0.05; $$, p < 0.01; $$$, p < 0.001; $$$$, p < 0.0001, GEE-treated vs. corresponding GEE-untreated group. ^, p < 0.05; ^^, p < 0.01; ^^^, p < 0.001, dnNrf2-HL60 cells vs. pEF-HL60 cells.
Figure 6.
Effects of GSH ethyl ester on mRNA levels of vitamin D- and Nrf2-related genes. pEF-HL60 and dnNrf2-HL60 cells were incubated with the indicated agents for 48 h. Samples were analyzed for mRNA levels of the indicated genes using quantitative RT-PCR. The expression of specific genes is normalized by the CT value of the internal reference gene (GAPDH). The data are means ± SD of 3 experiments performed in triplicate. *, p < 0.05; **, p < 0.01; ***, p < 0.001; ****, p < 0.0001 vs. corresponding untreated control group; #, p < 0.05; ##, p < 0.01; ###, p < 0.001; ####, p < 0.0001 vs. corresponding sum of the effects of single agents. $, p < 0.05; $$, p < 0.01; $$$, p < 0.001; $$$$, p < 0.0001, GEE-treated vs. corresponding GEE-untreated group. ^, p < 0.05; ^^, p < 0.01; ^^^, p < 0.001, dnNrf2-HL60 cells vs. pEF-HL60 cells.
Figure 7.
Effects of GSH ethyl ester on the levels of vitamin D- and Nrf2-related proteins, c-Jun and its phosphorylation. pEF-HL60 and dnNrf2-HL60 cells were incubated with the indicated agents for 48 h. Samples were analyzed for the expression of the indicated proteins by Western blotting. Calreticulin was used as a protein loading control. (a, j) Representative Western blot images. (b-i,k,l) Absorbance values for specific proteins normalized to those of calreticulin and expressed in the bar graphs as fold change relative to the corresponding untreated controls. The data are means ± SD of 3 experiments. *, p < 0.05; **, p < 0.01 vs. corresponding control group; #, p < 0.05; ##, p < 0.01; ###, p < 0.001 vs. corresponding sum of the effects of single agents; $, p < 0.05; $$, p < 0.01; $$$, p < 0.001, GEE-treated vs. corresponding GEE-untreated group; ^, p < 0.05; ^^, p < 0.01, dnNrf2-HL60 cells vs. pEF-HL60 cells.
Figure 7.
Effects of GSH ethyl ester on the levels of vitamin D- and Nrf2-related proteins, c-Jun and its phosphorylation. pEF-HL60 and dnNrf2-HL60 cells were incubated with the indicated agents for 48 h. Samples were analyzed for the expression of the indicated proteins by Western blotting. Calreticulin was used as a protein loading control. (a, j) Representative Western blot images. (b-i,k,l) Absorbance values for specific proteins normalized to those of calreticulin and expressed in the bar graphs as fold change relative to the corresponding untreated controls. The data are means ± SD of 3 experiments. *, p < 0.05; **, p < 0.01 vs. corresponding control group; #, p < 0.05; ##, p < 0.01; ###, p < 0.001 vs. corresponding sum of the effects of single agents; $, p < 0.05; $$, p < 0.01; $$$, p < 0.001, GEE-treated vs. corresponding GEE-untreated group; ^, p < 0.05; ^^, p < 0.01, dnNrf2-HL60 cells vs. pEF-HL60 cells.
Figure 8.
Involvement of AP-1 in the enhancing effects of Nrf2 activators on 1,25D3-induced cell differentiation, VDR and RXRα protein expression, and VDRE transactivation.HL60 cells were preincubated for 24 h either without oligodeoxynucleotide (ODN) or with 10 μM TRE-ODN or mTRE-ODN followed by treatment with 1,25D3, CA, MMF, or their combinations at the indicated concentrations for an additional 48 h. (a) Averaged CD14 and CD11b surface expression, as measured by flow cytometry. (b) Representative Western blots showing changes in VDR and RXRα protein levels. Calreticulin was used as a protein loading control. (c) Cells were transiently transfected with VDREx6-Luc and Renilla luciferase plasmids, followed by pretreatment with or without TRE-ODN or mTRE-ODN for 24 h. Cells were then incubated with or without the indicated test agents for another 24 h, followed by measuring luciferase activity. The data are means ± SD of 3 experiments. *, p < 0.05; **, p < 0.01; ***, p < 0.001; ****, p < 0.0001 vs. corresponding control group; #, p < 0.05; ##, p < 0.01; ###, p < 0.001; combination vs. corresponding sum of the effects of single agents; $$, p < 0.01, TRE-ODN-treated group vs. corresponding TRE-ODN untreated group.
Figure 8.
Involvement of AP-1 in the enhancing effects of Nrf2 activators on 1,25D3-induced cell differentiation, VDR and RXRα protein expression, and VDRE transactivation.HL60 cells were preincubated for 24 h either without oligodeoxynucleotide (ODN) or with 10 μM TRE-ODN or mTRE-ODN followed by treatment with 1,25D3, CA, MMF, or their combinations at the indicated concentrations for an additional 48 h. (a) Averaged CD14 and CD11b surface expression, as measured by flow cytometry. (b) Representative Western blots showing changes in VDR and RXRα protein levels. Calreticulin was used as a protein loading control. (c) Cells were transiently transfected with VDREx6-Luc and Renilla luciferase plasmids, followed by pretreatment with or without TRE-ODN or mTRE-ODN for 24 h. Cells were then incubated with or without the indicated test agents for another 24 h, followed by measuring luciferase activity. The data are means ± SD of 3 experiments. *, p < 0.05; **, p < 0.01; ***, p < 0.001; ****, p < 0.0001 vs. corresponding control group; #, p < 0.05; ##, p < 0.01; ###, p < 0.001; combination vs. corresponding sum of the effects of single agents; $$, p < 0.01, TRE-ODN-treated group vs. corresponding TRE-ODN untreated group.
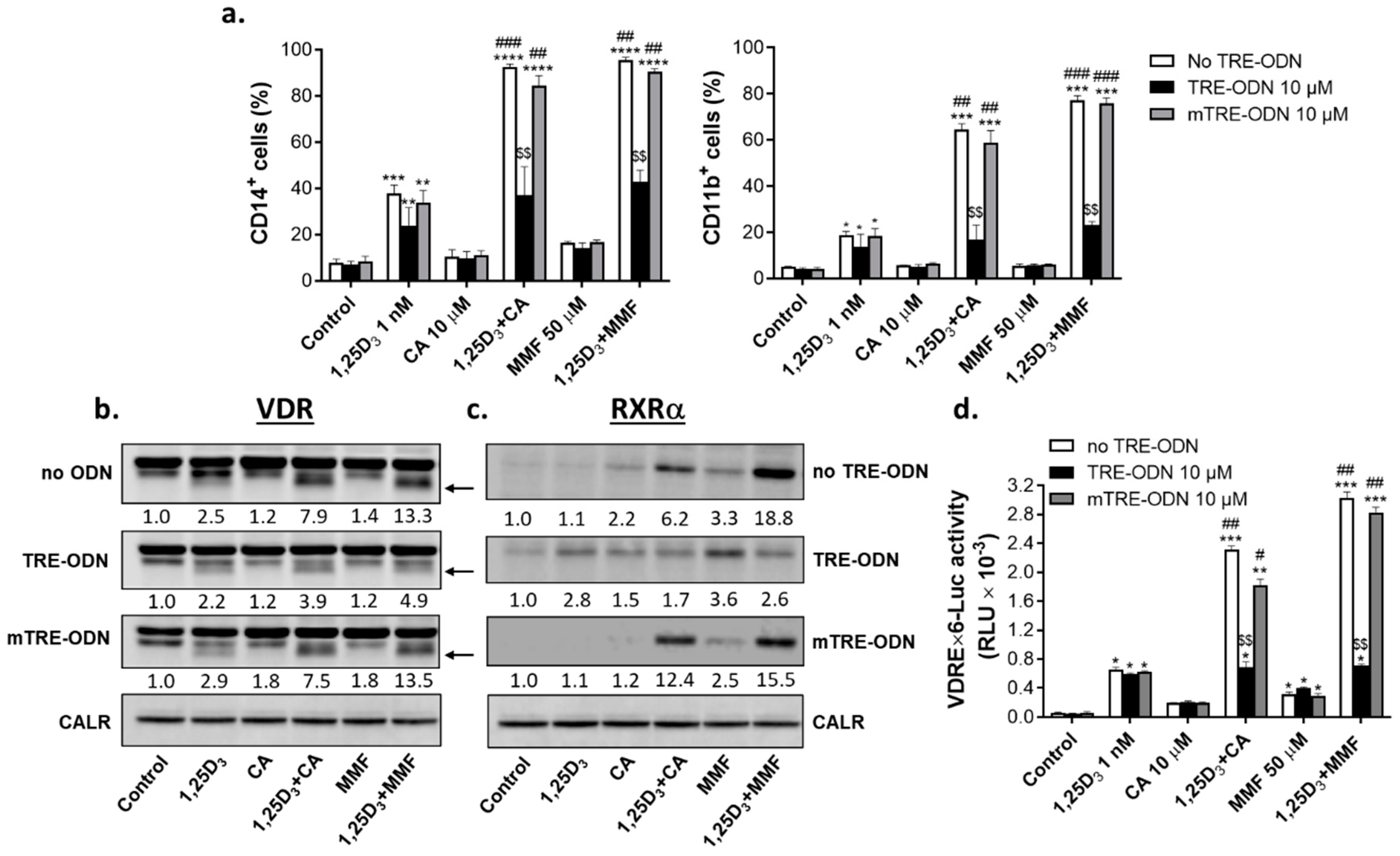
Figure 9.
Putative roles of glutathione and AP-1 in the cooperation between VDDs and Nrf2 activators in inducing differentiation of AML cells. See explanations in the text.
Figure 9.
Putative roles of glutathione and AP-1 in the cooperation between VDDs and Nrf2 activators in inducing differentiation of AML cells. See explanations in the text.