1. Introduction
Maize is a major cereal crop that serves as a significant source of food, feed, and industrial products around the world. Maize yield is significantly affected by insect herbivores, including fall armyworm (FAW), corn rootworms, aphids, and earworms [
1]. Among these insects, FAW [
Spodoptera frugiperda (J.E. Smith) (Insecta: Lepidoptera: Noctuidae)], is an especially highly destructive pest of not only maize but other crops like sorghum, rice, and other various grasses resulting in enormous economic losses worldwide [
2,
3]. Maize is one of the most widely grown staple food crops to many African communities, covering 37 million hectares in sub-Saharan Africa [
4,
5]. More than 300 million people in Africa are dependent on maize for food security [
6]. FAW is native to tropical and subtropical Americas, suddenly first arrived to Africa in 2016 and spread rapidly through the continent [
7,
8]. FAW remains an important pest in Africa’s farming systems, leading to maize yield losses up to 58% [
9,
10] and estimated annual economic losses amounting to
$9.4 billion in Africa alone [
9]. Therefore, there is an urgent need to develop maize germplasm that can resist damage from pests, especially for the countries that do not rely on transgenic crops containing
Bacillus thuringiensis (Bt)-genes.
The success of crop breeding programs aimed at developing varieties with superior resistance to insect herbivory is largely dependent on the
identification of novel defense-related alleles, introgression of which into elite germplasm does not result in the penalty for crop productivity. While it is well documented that plant resistance to chewing insects requires activation of jasmonic acid (JA) signaling pathways [
11,
12], breeding for increased production of JA does not represent a feasible strategy as increased levels of this defense hormone often results in growth inhibition [
13,
14]. Recently, we have identified a novel signaling pathway in maize that governs resistance to insect herbivory mediated by an oxylipin α-ketol, named 9,10-KODA [9-hydroxy-10-oxo-12(Z),15(Z)-octadecadienoic acid], which promotes plant herbivory resistance through defense priming and direct toxicity to FAW [
15,
16,
17]. This novel signaling molecule is the major product of a maize tonoplast-localized 9-lipoxygenase gene,
ZmLOX5 [
15,
17]. Mechanical wounding and insect feeding induce
ZmLOX5 expression and production of 9,10-KODA in a JA-dependent manner [
15,
18,
19]. The transposon-insertional disruption of
ZmLOX5 markedly reduced resistance to FAW and was accompanied by reduced levels of wound-induced 12-oxo-phytodienoic acid (12-OPDA), a JA-precursor and a hormone in its own right, JA, abscisic acid (ABA), green leaf volatiles (GLV) and insecticidal benzoxazinoids [
15]. Exogenous treatment of maize seedlings with 9,10-KODA specifically induced production of 12-OPDA and ABA, but not jasmonoyl-L-isoleucine (JA-Ile), and resulted in increased resistance to FAW [
15]. Hence,
ZmLOX5 represents a valid molecular breeding target for the development of maize lines that are more resistant to herbivory by chewing insects.
In addition to the identification of herbivory defense-related genes, another molecular breeding strategy is to exploit genome structure variations to identify superior loci of those genes. Genome structure variation mainly includes copy number variation (CNV) and/or presence/absence variation (PAV). Maize genome is characterized by extraordinarily high frequency of genetic diversity as assayed at the level of single nucleotide polymorphisms (SNPs), InDel polymorphisms and CNVs. The growing evidence indicates that CNVs are among the major reasons for maize phenotypic diversity [
20]. CNVs result from the genomic rearrangements that lead to gains or losses of DNA segments [
21,
22]. In our search for a superior
ZmLOX5 locus, we have sequenced around 1.3 Kb of the
ZmLOX5 gene spanning the last two exons in close to 400 inbred lines that represent maize diversity panel [
23]. Sequencing results indicated the presence of multiple SNPs and indels found in the
ZmLOX5 gene. Of direct releavance to this study, southern blotting analyses of 56 selected inbred lines identified several lines (including sweet, tropical, and temperate lines) that harbor duplicated copy variant of
ZmLOX5. This includes Yu796 inbred that we utilized in this study as a source of the duplicated locus, designated here as
Yu796-2×LOX5 [
23].
In this study, we determined that Yu796-2×LOX5 segregates as a single locus indicating that ZmLOX5 CNVs are tandemly duplicated. Next, we genetically introgressed the Yu796-2×LOX5 locus into the B73 background by 6 back-crosses (BC6) to B73-lox5-3 mutant previously generated in B73 background at the BC7 stage. The resulting B73-2×LOX5 lines are near-isogenic to B73 and contain either single or duplicated ZmLOX5 locus and were used to rigorously test whether increased copy number of this gene confers increased resistance to FAW. We found that maize resistance against FAW feeding was positively correlated with the gene dosage. Specifically, the seedlings homozygous for the duplicated ZmLOX5 (2×LOX5 / 2×LOX5, containing 4 copies present on the two homologous chromosomes displayed the greatest resistance, 2×LOX5 heterozygous seedlings (2 copies) displayed intermediate resistance and mutant seedlings lacking ZmLOX5 (B73-lox5-3 mutant locus, 0 copy) showed the least resistance. B73 inbred containing 2 ZmLOX5 copies exhibited resistance level similar to 2×LOX5 heterozygous seedlings (2 copies). We further showed that the duplication of ZmLOX5 resulted in increased wound-induced expression of ZmLOX5 and other defense-associated genes including ZmLOX8, ZmLOX10 and ZmJAR1a and increased wound-induced production of 9,10-KODA, 12-OPDA and ABA. Interestingly, the levels of the biologically active jasmonate, JA-Ile, were not significantly altered in B73-2×LOX5 lines compared to B73, likely due to the increased JA catabolism.
4. Discussion
The high level of structural variation with frequent changes in the genome content was observed in diverse maize genomes. When two maize genomes were compared, more than three thousand CNV or PNV sequences were identified [
20], while only several hundred CNV or PNV sequences were identified between the individual from human genomes using high resolution study [
40]. CNVs occur widely in plant genomes, however, only a few have been associated with obvious morphological, physiological or developmental phenotypes [
22,
41]. One of the possible explanations is that the paralogous genes in crops usually function redundantly. As a result, the difference in copy number of one gene may lead to a change in quantitative traits, but not in the variation of qualitative traits. We observed similar results in this study. The variance of copy number of
ZmLOX5 quantitatively affected maize anti-insect resistance phenotype in a step-wise gene-dosage dependent manner . Seedlings containing 4 copies of
ZmLOX5 displayed the strongest insect resistance, heterozygous for
2×LOX5 (
2×LOX5 /
lox5-3) seedlings or B73 that contain 2 functional copies of
ZmLOX5 displayed intermediate level of resistance, and the homozygous
lox5-3 mutant displayed the least insect resistance. It is important to emphasize that th eB73 line harboring duplicated
ZmLOX5 CNVs was sigificantly more resistant to FAW compared to B73 inbred line, which contains a single copy of
ZmLOX5. The increased resistance to FAW is due to increased expression of
ZmLOX5, which in turn resulted in increased production of the major ZmLOX5 product, 9,10-KODA, that we previously showed to possess a potent signaling activity in the activation of maize defense against insect herbivores [
15]. Here, we showed that the increased accumulation of 9,10-KODA was accompanied by the increased content of wound-induced levels of 12-OPDA and ABA. These results agree well with our previous study that showed that the
lox5 knock-out mutants, in addition to reduced 9,10-KODA levels, displayed reduced levels of 12-OPDA and ABA, the two defense hormones regulated by 9,10-KODA since both of them were strongly induced by exogenous application of this α-ketol [
15].
Another consistant observation is that the increased ZmLOX5-mediated herbivore defense does not result from increased JA production. Previously, we showed that ZmLOX5 acts downstream of JA in defense since the treatment with exogenous JA did not rescue susceptibility of
lox5 mutants. Moreover, exogenous 9,10-KODA suppresed wound-induced prodcution of JA-Ile. Here, we present evidence that the lack of increased wound-induced JA production in B73-2xLOX5 can be explained by increased rate of JA catabolism . The significance of JA catabolism in the dowregulation of the levels of the biologically active JA-Ile is best illustrated by the recent study of maize
Tasselseed5 (
Ts5) mutant. This mutant displayed a JA-deficiency phenotype, including
tasselseed and reduced wound-induced JA production [
35]. Positional cloning and transcriptomics analysis revealed that
ZmCYP94B1 is upregulated in
Ts5 and this gene regulated ω-oxidation of JA to convert JA or JA-Ile to 12OH-JA or 12OH-JA-Ile, respectively, resulting in the reduced JA accumulation [
35]. The disruption of
12-oxo-phytodienoic acid reductases2 (
ZmOPR2) reduced wound-induced JA and hebivory defense to FAW associated with increased JA catabolism [
36]. Consitant with these studies, our data showed that the wound-induced productions of 12OH-JA, 12OH-JA-Ile and 12COOH-JA-Ile were significantly enhanced in B73-
2×LOX5 seedlings compared to B73 inbred line, suggesting that ZmLOX5-mediated pathway promote JA catabolism through as yet unknown mechanism.
Another unanticipated finding in this study is that the duplication of ZmLOX5 contributes to plant drought tolerance presumably due to increased ABA and 12-OPDA production. ABA is well-documented to enhance drought tolerance in plant through the regulatory of stomatal closure to reduce water loss by reducing transpiration rate [
38,
42,
43], and also increaing evidence revealed that 12-OPDA is involved in stomatal movement [
39,
44]. Our previous study revealed that exogenous apllication of 9,10-KODA resulted in increased ABA and 12-OPDA accumulation [
15]. It is consistent with another study that showed 9,10-KODA treatment enhanced drought tolerance in wheat [
45].
While our study provides evidence that CNVs of certain defense genes can be utilized for improving herbivory defense and drought tolerance, previous studies showed that CNVs contribute to enhancements of other plant traits. For example, increased gene copy number lead to improved resistance against soybean cyst nematodes [
41]. Also, a recent review identified numerous examples of gene dosage that emerged in the course of plant adaptation to stressful environments and resulted in altered traits such as enhanced cold tolerance in grasses, and enhanced herbicide resistance in weeds [
46]. In maize, gene duplication effects on shaping certain traits have also been reported [
47,
48,
49,
50,
51]. For example, maize plants containing a duplicated 14.6-Mb segment of chromosome 1 showed dosage-dependent effects on ear length and flowering time [
48]. CNV analysis by whole genome sequencing of the lines highly resistant or highly susceptible to Goss’ bacterial wilt revealed structural genomic differences in 141 genes including CNVs. One such CNV gene was the
rp1 rust resistance locus likely involved in resistance to this pathogen [
52]. Additionally, maize plants with duplicated copies of the
tb1-ref gene displayed increases in crown root number, and increased density of first- and second-order lateral roots [
50]. Duplication at the 27-kDa γ-zein locus resulted in enhanced expression of γ-zein protein, which in turn led to endosperm modification from chalky to vitreous, yielding quality protein maize (QPM) [
51]. In addition to quantitative traits, the increased gene copy number also contributes to qualitative phenotypic traits. For example, pod corn (
Tunicate maize) is the result of dominant gain-of-function mutation at the
Tunicate (Tu) locus. The wild-type locus contains a single-copy gene that is only expressed in leaf tissue, however duplication of
ZMM19 in
Tu locus resulted in ectopic expression of the gene in the inflorescences, thereby conferring vegetative traits to the reproductive organ [
53]. Overall, our study provided much needed proof-of-concept that it is possible to substantially improve maize resistance by the genetic introgression of the duplicated CNVs of
ZmLOX5 into elite germplasm that is susceptible to herbivory such as B73.
An important aspect of this study is that we successfully adopted ddPCR technology for the accurate estimation of CNVs. While the original discovery of the duplicated
ZmLOX5 was based on Southern blotting method, this method is not applicable for molecular breeding programs that rely on genotyping large numbers of individuals at each pollination event.
Here, we used ddPCR to confirm the presence of duplicated ZmLOX5 CNVs in Yu796, the original source of the 2×ZmLOX5 locus, and in B73-2×ZmLOX5 populations. ddPCR
is able to accurately estimate copy number of specific DNA fragments by dividing a PCR reaction into thousands of nanoliter-scale droplets, so that presence or absence of sequence of interest in a droplet is determined by end-point fluorescence, which can be digitally counted [
28]
. Previously, ddPCR has been mainly used to accurately determine the number of transgenes in plants [
25,
30]
.
Figure 1.
Digital droplet PCR identification of copy number variation of ZmLOX5 in B73 (1×LOX5), Yu796 (2×LOX5), B73-2×LOX5 genetic backgrounds of maize. B73 inbred line carries 2 functional copies of the ZmLOX5 gene on the two homologous chromosomes, Yu796 inbred line carries 4 functional copies of ZmLOX5 (designated as 2×LOX5) and three independent near-isogenic lines of B73 carrying 4 functional copies of ZmLOX5 gene from Yu796, designated B73-2×LOX5, at the BC6F3 genetic stage. House-keeping single-copy gene, α-Tubulin (Zm00001eb215710), was chosen as an internal reference. A, one-dimensional plots of droplets measured for fluorescence signal (amplitude indicated on y-axis) emitted from the droplets containing either ZmLOX5 or α-Tubulin from each individual. Evergreen™-bound gene-positive droplets are shown in blue, while negative droplets are shown in grey. Top panel, fluorescence amplitudes of the target gene, ZmLOX5; bottom panel, amplitudes of the reference gene, α-Tubulin. B, template concentration (copies / μl) of the target gene, ZmLOX5 (black bars), and reference gene, α-Tubulin (grey bars) in B73 and Yu796 inbred lines, and in three independent near-isogenic lines B73-2×LOX5, determined by ddPCR. C, calculated ZmLOX5 copy numbers using the ratio of the concentrations of the target gene and reference gene (ZmLOX5/α-Tubulin).
Figure 1.
Digital droplet PCR identification of copy number variation of ZmLOX5 in B73 (1×LOX5), Yu796 (2×LOX5), B73-2×LOX5 genetic backgrounds of maize. B73 inbred line carries 2 functional copies of the ZmLOX5 gene on the two homologous chromosomes, Yu796 inbred line carries 4 functional copies of ZmLOX5 (designated as 2×LOX5) and three independent near-isogenic lines of B73 carrying 4 functional copies of ZmLOX5 gene from Yu796, designated B73-2×LOX5, at the BC6F3 genetic stage. House-keeping single-copy gene, α-Tubulin (Zm00001eb215710), was chosen as an internal reference. A, one-dimensional plots of droplets measured for fluorescence signal (amplitude indicated on y-axis) emitted from the droplets containing either ZmLOX5 or α-Tubulin from each individual. Evergreen™-bound gene-positive droplets are shown in blue, while negative droplets are shown in grey. Top panel, fluorescence amplitudes of the target gene, ZmLOX5; bottom panel, amplitudes of the reference gene, α-Tubulin. B, template concentration (copies / μl) of the target gene, ZmLOX5 (black bars), and reference gene, α-Tubulin (grey bars) in B73 and Yu796 inbred lines, and in three independent near-isogenic lines B73-2×LOX5, determined by ddPCR. C, calculated ZmLOX5 copy numbers using the ratio of the concentrations of the target gene and reference gene (ZmLOX5/α-Tubulin).
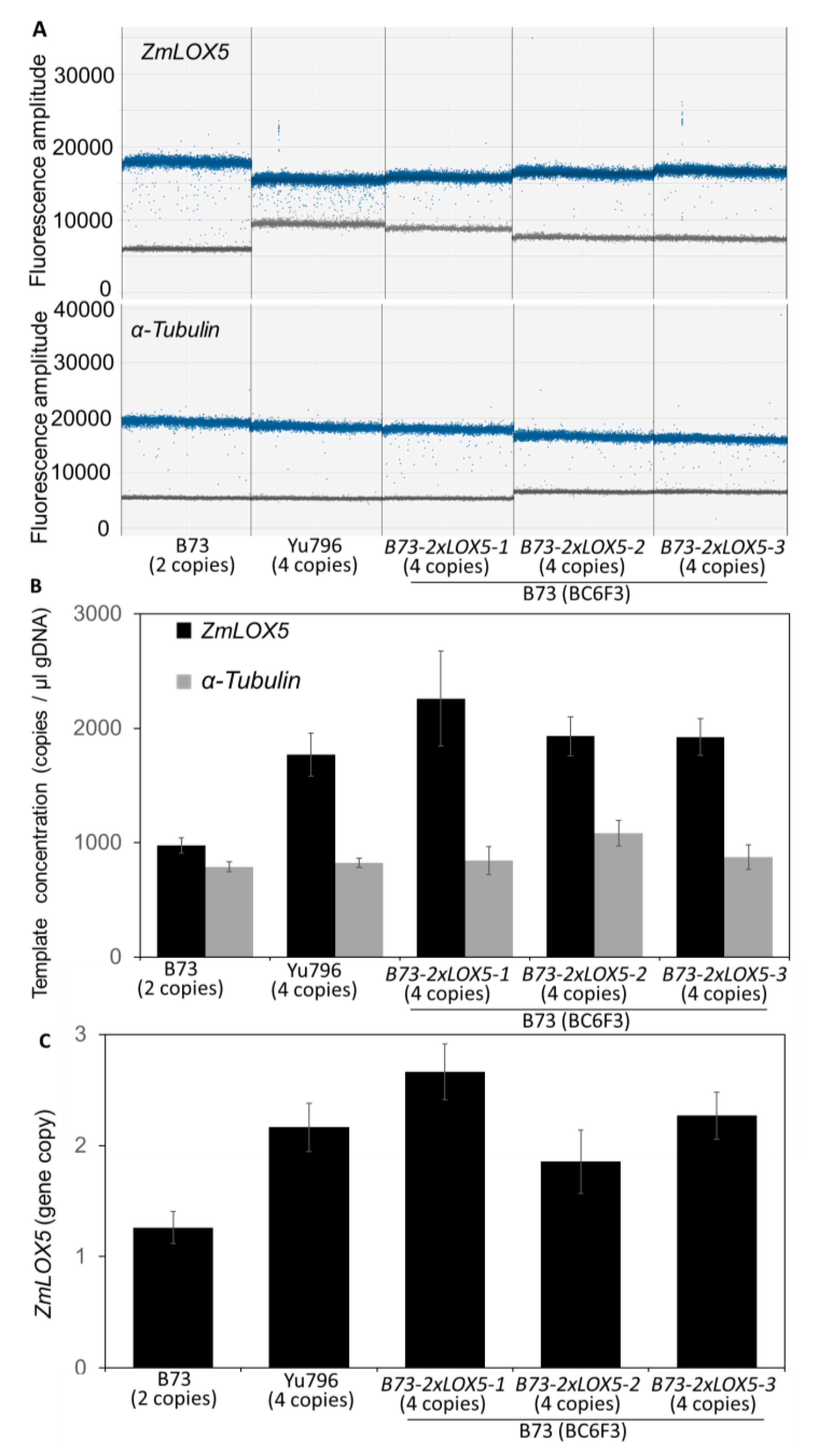
Figure 2.
Alignment of the nucleotide sequences of the 8th intron of the ZmLOX5 gene in B73 and the copy number variants (CNV) of ZmLOX5 in Yu796 (Yu796-CNV1 and Yu796-CNV2). Upper panel: genomic structure of the ZmLOX5 gene containing 9 exons (shown in blue color), 8 introns (shown in black color), and 5’-UTR and 3’-UTR (shown in green color). ZmLOX5-specific primers gDL-F and gDL-R (shown in red color) cover partially exon VIII, complete exon IX and 3’UTR, and complete 8th intron. Sequences highlighted in yellow color represent the nucleotide differences between the B73-LOX5 and Yu796-2×LOX5 locus. Nucleotides shown in red color represents the difference between the Yu796-CNV1 and Yu796-CNV2 locus.
Figure 2.
Alignment of the nucleotide sequences of the 8th intron of the ZmLOX5 gene in B73 and the copy number variants (CNV) of ZmLOX5 in Yu796 (Yu796-CNV1 and Yu796-CNV2). Upper panel: genomic structure of the ZmLOX5 gene containing 9 exons (shown in blue color), 8 introns (shown in black color), and 5’-UTR and 3’-UTR (shown in green color). ZmLOX5-specific primers gDL-F and gDL-R (shown in red color) cover partially exon VIII, complete exon IX and 3’UTR, and complete 8th intron. Sequences highlighted in yellow color represent the nucleotide differences between the B73-LOX5 and Yu796-2×LOX5 locus. Nucleotides shown in red color represents the difference between the Yu796-CNV1 and Yu796-CNV2 locus.
Figure 3.
Duplication of ZmLOX5 in the B73 background results in enhanced expression of ZmLOX5 and selected 12-OPDA and JA biosynthesis genes in response to wounding compared to the B73 near isogenic line containing a single gene. RT-qPCR analysis of wound-induced gene expression of ZmLOX5, ZmLOX8, ZmLOX10 and ZmJAR1a in B73 line and in the near-isogenic lines containing duplicated ZmLOX5 CNVs in the B73 genetic background (B73-2×LOX5) at the BC6F3 stage at 0, 1, 2 and 4 hours post wounding (hpw). The 2-ΔΔCt method was used with α-tubulin utilized as the house-keeping gene and compared to the mean at 0 h. For each graph, the X-axis represents hpw while the Y-axis represents expression fold change; black line represents the B73-2×LOX5 line (4 copies of ZmLOX5) and green line represents B73 (2 copies of ZmLOX5), bars are means ± SE; n=4. Asterisks represent significant differences between B73-2×LOX5 and B73 lines at each time point using Student’s t-test (*p < 0.05 and **p < 0.01).
Figure 3.
Duplication of ZmLOX5 in the B73 background results in enhanced expression of ZmLOX5 and selected 12-OPDA and JA biosynthesis genes in response to wounding compared to the B73 near isogenic line containing a single gene. RT-qPCR analysis of wound-induced gene expression of ZmLOX5, ZmLOX8, ZmLOX10 and ZmJAR1a in B73 line and in the near-isogenic lines containing duplicated ZmLOX5 CNVs in the B73 genetic background (B73-2×LOX5) at the BC6F3 stage at 0, 1, 2 and 4 hours post wounding (hpw). The 2-ΔΔCt method was used with α-tubulin utilized as the house-keeping gene and compared to the mean at 0 h. For each graph, the X-axis represents hpw while the Y-axis represents expression fold change; black line represents the B73-2×LOX5 line (4 copies of ZmLOX5) and green line represents B73 (2 copies of ZmLOX5), bars are means ± SE; n=4. Asterisks represent significant differences between B73-2×LOX5 and B73 lines at each time point using Student’s t-test (*p < 0.05 and **p < 0.01).
Figure 4.
Increased gene dosage of ZmLOX5 results in increased resistance to fall armyworms (FAW). A and B. Effect of ZmLOX5 duplication on FAW resistance during localized herbivory in the homozygous seedlings (2×LOX5/2×LOX5, 4 copies), heterozygous seedlings (2×LOX5/lox5-3, 2 copies), knockout mutants (lox5-3/lox5-3, 0 copy) and B73 (2 copies). Second to third instar larvae were confined to clip-cages positioned on the maize leaf and allowed to feed for 6-8 h. Area consumed was determined by ImageJ analysis of scanned leaves. (C-F). The individual seedlings as in A and B were exposed to continued feeding by 8 neonates per seedling for 7 days. Afterwards, seedlings were photographed (C) and weighed (D), bars are means ± SE; n=6-7; the recovered larvae were photographed (E), and the fresh weight of recovered larvae were weighed (F). Bars are means ± SE; n=16-20. Groups with non-overlapping letters represent statistically significance differences (p<0.05) by one-way ANOVA with Tukey HSD post-hoc test.
Figure 4.
Increased gene dosage of ZmLOX5 results in increased resistance to fall armyworms (FAW). A and B. Effect of ZmLOX5 duplication on FAW resistance during localized herbivory in the homozygous seedlings (2×LOX5/2×LOX5, 4 copies), heterozygous seedlings (2×LOX5/lox5-3, 2 copies), knockout mutants (lox5-3/lox5-3, 0 copy) and B73 (2 copies). Second to third instar larvae were confined to clip-cages positioned on the maize leaf and allowed to feed for 6-8 h. Area consumed was determined by ImageJ analysis of scanned leaves. (C-F). The individual seedlings as in A and B were exposed to continued feeding by 8 neonates per seedling for 7 days. Afterwards, seedlings were photographed (C) and weighed (D), bars are means ± SE; n=6-7; the recovered larvae were photographed (E), and the fresh weight of recovered larvae were weighed (F). Bars are means ± SE; n=16-20. Groups with non-overlapping letters represent statistically significance differences (p<0.05) by one-way ANOVA with Tukey HSD post-hoc test.
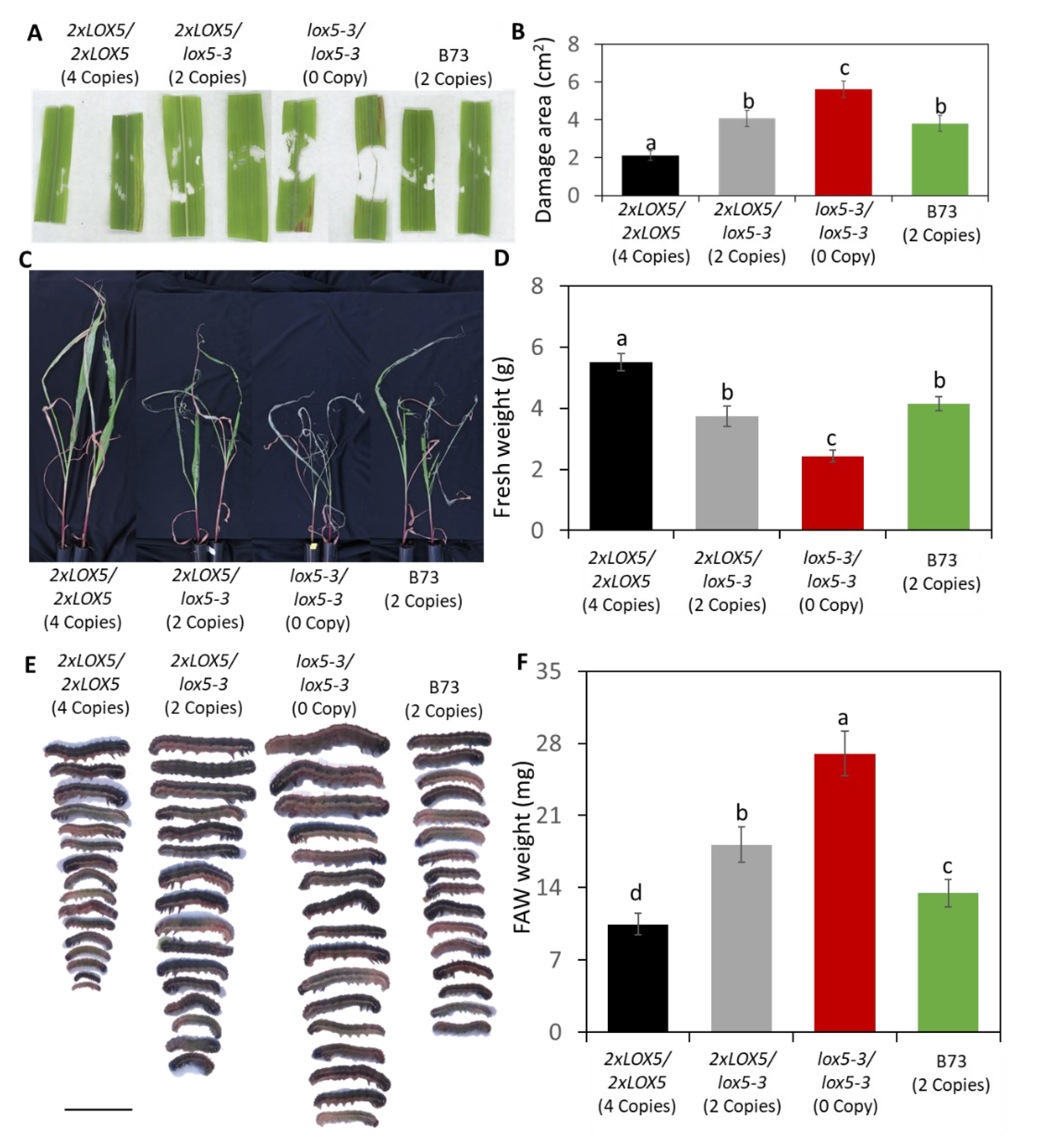
Figure 5.
Duplication of ZmLOX5 enhanced 9,10-KODA, 12-OPDA, and ABA, but not JA-Ile, accumulation in response to mechanical wounding. For each graph, the X-axis represents hpw and the Y-axis represents metabolite concentration in pmol/g FW for each metabolite (JA-Ile, 12-OPDA, 9,10-KODA and ABA) indicated within the graphs. Black line represents duplicated copy variant (2×ZmLOX5/2×ZmLOX5, 4 copies of ZmLOX5 in the B73 genetic background at the BC6F3 genetic stage) and green line represents B73 inbred line (2 copies of ZmLOX5); bars are means ± SE; n=4-5; asterisks represent significant differences near-isogenic 2×LOX5 and B73-LOX5 at each time point using Student’s t-test (*p < 0.05 and **p < 0.01).
Figure 5.
Duplication of ZmLOX5 enhanced 9,10-KODA, 12-OPDA, and ABA, but not JA-Ile, accumulation in response to mechanical wounding. For each graph, the X-axis represents hpw and the Y-axis represents metabolite concentration in pmol/g FW for each metabolite (JA-Ile, 12-OPDA, 9,10-KODA and ABA) indicated within the graphs. Black line represents duplicated copy variant (2×ZmLOX5/2×ZmLOX5, 4 copies of ZmLOX5 in the B73 genetic background at the BC6F3 genetic stage) and green line represents B73 inbred line (2 copies of ZmLOX5); bars are means ± SE; n=4-5; asterisks represent significant differences near-isogenic 2×LOX5 and B73-LOX5 at each time point using Student’s t-test (*p < 0.05 and **p < 0.01).
Figure 6.
JA catabolites, 12OH-JA, 12OH-JA-Ile and 12COOH-JA-Ile, were significantly increased in the B73-2×LOX5 seedlings in response to mechanical wounding. A. (A) Heatmap showing relative accumulation of 9-oxylipins, 13-oxylipins and ABA in B73-2×LOX5, duplicated copy variant (2×ZmLOX5/2×ZmLOX5, 4 copies of ZmLOX5 in the B73 genetic background at the BC6F3 genetic stage) and B73 inbred line (2 copies of ZmLOX5) at 1, 2 and 4 h post wounding (hpw) compared to unwounded B73. Cells are shaded by Z-score scaling within columns, with the higher-abundant metabolite in red and the lower-abundant metabolite in blue; B. For each graph, the X-axis represents hpw and the Y-axis represents metabolite concentration in pmol / g FW for each JA catabolites (12OH-JA, 12OH-JA-Ile and 12COOH-JA-Ile) indicated within the graphs. Black line represents duplicated copy variant (2×ZmLOX5/2×ZmLOX5, 4 copies of ZmLOX5 in the B73 genetic background at the BC6F3 genetic stage) and green line represents B73 inbred line (2 copies of ZmLOX5); bars are means ± SE; n=4-5; asterisks represent significant differences near-isogenic 2xLOX5 and B73-LOX5 at each time point using Student’s t-test (*p < 0.05 and **p < 0.01).
Figure 6.
JA catabolites, 12OH-JA, 12OH-JA-Ile and 12COOH-JA-Ile, were significantly increased in the B73-2×LOX5 seedlings in response to mechanical wounding. A. (A) Heatmap showing relative accumulation of 9-oxylipins, 13-oxylipins and ABA in B73-2×LOX5, duplicated copy variant (2×ZmLOX5/2×ZmLOX5, 4 copies of ZmLOX5 in the B73 genetic background at the BC6F3 genetic stage) and B73 inbred line (2 copies of ZmLOX5) at 1, 2 and 4 h post wounding (hpw) compared to unwounded B73. Cells are shaded by Z-score scaling within columns, with the higher-abundant metabolite in red and the lower-abundant metabolite in blue; B. For each graph, the X-axis represents hpw and the Y-axis represents metabolite concentration in pmol / g FW for each JA catabolites (12OH-JA, 12OH-JA-Ile and 12COOH-JA-Ile) indicated within the graphs. Black line represents duplicated copy variant (2×ZmLOX5/2×ZmLOX5, 4 copies of ZmLOX5 in the B73 genetic background at the BC6F3 genetic stage) and green line represents B73 inbred line (2 copies of ZmLOX5); bars are means ± SE; n=4-5; asterisks represent significant differences near-isogenic 2xLOX5 and B73-LOX5 at each time point using Student’s t-test (*p < 0.05 and **p < 0.01).
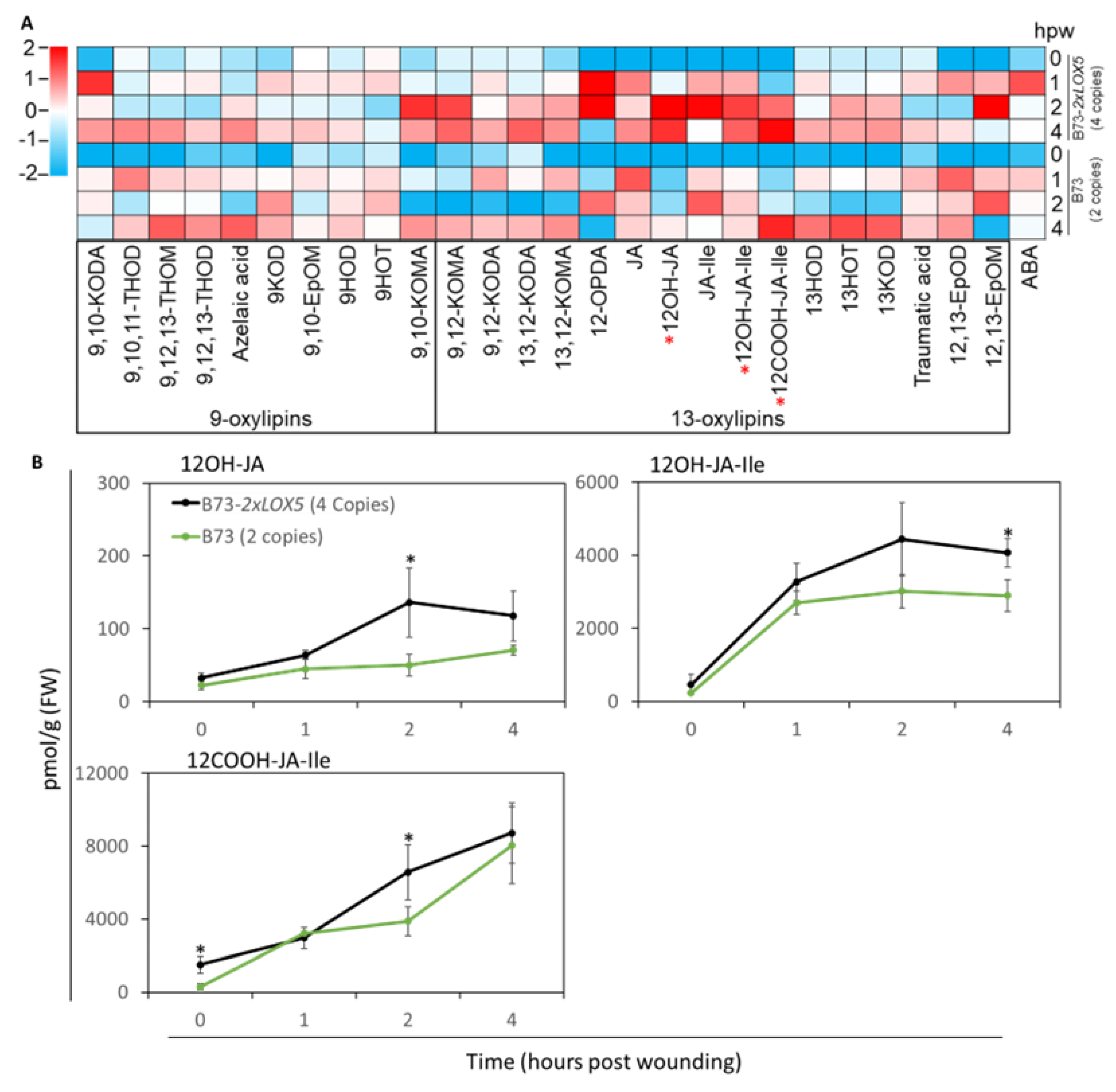
Table 1.
Duplicated copy variant of ZmLOX5 (2×LOX5) segregates in the mendelian ratio consistent with a single locus, confirming that the two copies are linked as tandem duplicates, designated as copy number variant 1 (LOX5-CNV1) and 2 (LOX5-CNV2).
Table 1.
Duplicated copy variant of ZmLOX5 (2×LOX5) segregates in the mendelian ratio consistent with a single locus, confirming that the two copies are linked as tandem duplicates, designated as copy number variant 1 (LOX5-CNV1) and 2 (LOX5-CNV2).