1. Introduction
Parkinson’s disease (PD) is a major neurodegenerative disease that causes motor dysfunction in 1% of the population over 65 years [
1]. Traditionally, PD has been considered a motor disorder, and it is clinically diagnosed based on the presence of bradykinesia with at least one of resting tremor, rigidity, or postural instability [
2]. The motor dysfunction is largely due to dopaminergic neuronal loss in the
Substantia nigra pars compacta (SNpc) [
3]. However, extranigral changes in the central nervous system and also in the enteric and peripheral nervous systems likely contribute to the numerous non-motor symptoms observed in PD [
4]. A wide range of non-motor PD features, such as cognitive impairment, hallucinations, autonomic dysfunction, restless leg syndrome, disorders of sleep, and depression are prevalent. Among these, the main non-motor symptoms frequently encountered include hyposmia and gastrointestinal (GI) dysfunction [
3]. Impairments of peripheral sensory function also occur in PD and indeed, disorders of skin are more predictive of subsequent PD diagnosis than even olfactory deficits [
5]. Moreover, aggregates of alpha-synuclein are observed in skin and are similar to those observed in the brain [
6], and the seeding capacity of PD-patient-skin-derived alpha-synuclein may be used for antemortem diagnosis of PD and other synucleinopathies[
7]. Critically, peripheral neuropathy may be considered unrelated to PD by patients and is therefore likely underdiagnosed in PD patients [
8], and indeed, treatments for non-motor symptoms in PD are a priority for development.
Peripheral neuropathy (PN) is a general term that indicates any dysfunction in the sensory, motor, and autonomic nerves of the peripheral nervous system [
9]. PN can also manifest as postural instability, loss of peripheral sensation, weakness, and/or pain, and it can be divided into small- (unmyelinated C fibers and thinly myelinated A δ fibers) and large-fiber neuropathy [
10].
The cause of PD in the majority of patients is unknown but several genetic risk factors have now been characterized, as well as several genes that cause rare autosomal dominant forms of PD [
3]. Environmental factors, such as well-derived water, pesticides, herbicides, and farming activities have also been reported as significant factors in causing PD in men [
11]. Importantly, agricultural chemicals, such as rotenone and paraquat, which are pesticides and piscicides, have been shown to cause dopaminergic cell death in vivo and in vitro and chronic exposure increases the risk of PD in humans [
12,
13].
Rotenone is a validated risk factor for PD [
13]. It is a naturally occurring insecticide, pesticide, and piscicide extracted from the roots of plants of the genera
Lonchocarpus and
Derris. It is highly lipophilic and therefore easily crosses all biological membranes including the blood-brain barrier [
12]. It impairs oxidative phosphorylation by inhibiting mitochondrial electron transport chain complex I (ubiquinone oxidoreductase), leading to reduced ATP production and the formation of reactive oxygen species that can induce oxidative stress [
14,
15]. A major advantage of rotenone in modeling PD is that upon chronic ingestion of rotenone, alpha-synuclein accumulates in the enteric nervous system (ENS), which then induces transneuronal distribution of misfolded alpha-synuclein to the hind- (dorsal motor nucleus of the vagus) and midbrain (SNpc) of experimental animals [
16,
17,
18]. Animal models exposed to rotenone and paraquat have received the most attention because chronic exposure increases the risk of PD in agricultural workers [
13].
Several articles have been published where rotenone was administered intrastriatally (unilateral) to reproduce the neuropathological signs of PD, including dopaminergic cell loss in the SNpc [
12,
19]. This model has been shown to develop bilateral loss of tyrosine hydroxylase-positive neurons in SNpc and motor impairment [
20]. Interestingly, this model suggested possible bidirectional communication between the gut and the brain, suggesting that it could induce other peripheral signs, including sensory neuropathy. However, here, we report no changes in non-motor signs, using gold-standard, statistically well-powered protocols that were tested repeatedly. Non-motor signs that were tested included whole-gut transition, and sensitivity to heat, cold and touch. Given that most preclinical trials of therapeutics use MPTP or 6-OHDA [
21], which cause specific lesions of the SNpc, our data show that in mice, degeneration of TH-positive neurons in SNpc alone is not sufficient to reproduce the full spectrum of PD.
2. Materials and Methods
2.1. Animals
Male C57Bl/6N mice, initially aged 84 days and weighing 24–29 g (n = 22), were used in this study. The animals were housed at 21-23 °C under a 12 hours light-dark cycle (7am on and 7pm off) with free access to ad lib food (V1534-300, ssniff Spezialdiäten GmbH) and water (reverse osmosis-treated, and UV sterilised). The experimental procedures were carried out in accordance with the Estonian Animal Welfare Authorisation Committee (#198) according to the ARRIVE guidelines [
22] and EU Directive 2010/63/EU.
2.2. Stereotaxic Surgery
Before the stereotaxic surgery, animals were divided into control and treated groups based upon rotarod and grip-strength performance during baseline training (see below). Two additional animals were used to confirm injection site. For stereotaxic microinjections, mice were anaesthetised using 2% isoflurane in oxygen and the skin overlying the skull was sterilized for surgery. Following placement in the stereotaxic frame, anaesthesia was maintained using approximately 1.5% isoflurane in oxygen. Ophthalmic ointment was placed on eyes, and rectal temperature and respiration rate were monitored throughout. An incision was made in the skin overlying the skull and coordinates at the bregma and at AP+2mm and AP-2mm were obtained. The skull was then adjusted until the skull surface was flat (tolerance <50µm). Mice were treated with 5.4µg of rotenone in 2µL DMSO (N=10) or vehicle only (DMSO N=12) at the following coordinates: AP: + 0.4mm; ML: -2.5mm and DV: -3.5mm, which causes bilateral loss of TH-positive neurons in [
20]. Solutions were carefully protected from light. A Hamilton syringe (Hamilton Americas& Pacific Rim, Hamilton Company Inc) was used to deliver rotenone or vehicle over a period of 20 minutes (approximately 100nl per minute). The needle was left in place for 2 minutes and then withdrawn over a further 2 minutes. Each mouse was administered with 0.5ml saline (subcutaneous, sc) and carprofen (5mg/kg sc) during the surgery to ensure appropriate hydration and analgesia during recovery. The surgery procedure took approximately 1 hour/mouse. Following suturing, mice were placed into a heated cage with food and water, for approximately 1 hour before returning them to their home cage. Analgesia was administered at regular intervals until 24 hours after surgery.
2.3. General Health and Motor Testing
Mice were monitored daily and weighed every two days.
2.3.1. Open Field
Spontaneous activity in a novel environment, over a period of 1 hour, was analysed as per [
23] at baseline and at 5, 10 and 15 weeks post surgery.
2.3.2. Grip Strength
The mouse was placed onto the grip strength meter (TSE Systems, Germany) and then encouraged to grip by pulling gently on the tail. Forelimb and all-four-limb grip strength was measured at baseline and at 5, 10 and 15 weeks after surgery. Mice were given 5 trials and the best three trials were used for analysis.
2.3.3. Rotarod
An accelerating protocol was used, as per [
24], as this is more sensitive to striatal impairment. All animals were pre-trained on the rotarod apparatus, comprising three trials per day over 4 consecutive days, with at least 20 minutes of rest between trials. Following surgery, rotarod performance was evaluated every two weeks (four trials over a period of 1 day, except in the case of week 2 where mice completed four trials per day over a period of 2 days).
2.3.4. Hindlimb Stepping
Testing was done as per [
25] at baseline and at 5, 10 and 15 weeks post surgery. Importantly, levodopa administration improves performance in this task in unilateral 6-OHDA-lesioned mice [
26], showing its superior sensitivity and specificity for models of PD.
2.3.5. Gait Test
Footprinting was used to compare the gait of rotenone-treated mice with control mice, at the final time point (week 15) only. Hind- and forefeet of the mice were coated with green and blue non-toxic paints, respectively [
27]. The animals were then allowed to walk along a 1-m long, 10-cm wide runway (with 10-cm high walls) into an enclosed chamber. All mice had training runs prior to the run used for analysis, although extra runs were used if footprints were indistinct. The footprint patterns were analysed for stride width, length and overlap as per [
27].
2.4. Gut Motility
Colonic motility was examined by quantifying faecal pellet output over 8-hour period following gavage with carmine red [
28], which is not absorbed from the lumen of the gut. Carmine red (300 µL per mouse; 6%; Sigma-Aldrich, Germany) was suspended in 0.5% methylcellulose (Sigma-Aldrich, Germany) and administered by oral gavage. Before administering, each animal was placed in an individual cage, with some food and water. The number of regular and of red faecal pellets was counted at 60-minutes intervals for up to 8 hours. GI motility was examined at baseline and at 5, 10 and 15 weeks after surgery.
2.5. Sensory Testing
Behavioural responses of rodents during hot-plate and cold-plate protocols are highly variable [
29]. Thus, these protocols were not used. Importantly, for the Hargreaves, cold-plantar and von Frey tests, mice were habituated to their test environment for 1 hour [
29]. Mice that were grooming or sleeping were not tested – the personnel simply moved onto the next available mouse and returned at a later time during the session. For other tests, mice were habituated to the testing room for 30 minutes.
2.5.1. Hargreaves Test
The Hargreaves test (UGO Basile) (intensity IR: 50) was carried out as per review [
29]. Gently, each animal was placed into its own compartment with a glass floor and habituated for 1 hour. The latency to remove the paw from the heat source over three trials was measured automatically, at baseline and at 5, 10 and 15 weeks post surgery.
2.5.2. Tail Flick Test
The time taken for mice to flick their tail away from the heat source, over two trials, was measured automatically (Ugo Basile, Italy) at baseline and at 5, 10 and 15 weeks post surgery. An IR heat intensity of 50 was used because preliminary testing revealed that lower intensities of 15, for example, resulted in latencies similar to “no intensity”.
2.5.3. Cold Plantar Test
This test was conducted at weeks 10 and 15, and each hind-paw was tested twice within each session. Gently, each animal was placed into its own compartment with a glass floor and habituated for 1 hour [
29,
30]. The flat end of a dry-ice pellet was applied to the glass surface underneath the paw of the mouse and latency to withdrawal was measured with a stopwatch (two timers controlled by two separate experimenters). Withdrawal was defined as any action to move the paw vertically or horizontally away from the cold site. An interval of at least 7 minutes was allowed between testing separate paws on a single mouse, and an interval of at least 15 minutes was allowed between trials on any single paw. The maximum time allowed for withdrawal was 20 seconds to avoid potential tissue damage.
2.5.4. Hot- and Cold-Exploration Test
Empiric preference for heat or cold were assessed at weeks 10 and 15. Animals were allowed to freely explore an open arena (100x10x20 cm) over a period of 30 minutes and their activity recorded using Ethovision XT V8 (Noldus, Wageningen, Netherlands).The arena comprised a glass floor, placed upon a hot plate at one end and ice packs at the other end. Preliminary experiments showed that floor temperatures ranged from 32°C on one side to 10 °C on the other side and that temperatures remained constant over a period of 30 minutes. The proportion of time spent in each temperature zone was analysed automatically by Ethovision.
2.5.5. Von Frey Test
In this test, each animal was placed into its own compartment and habituated for 1 hour [
29]. A monofilament was applied perpendicularly to the plantar surface of the hind paw until it buckled or the mouse responded. A response was considered positive if the animal exhibited any nocifensive behaviours, e.g., brisk paw withdrawal during application of the stimulus. The applied force began at 0.16g and testing followed the up-down method for greatest sensitivity and the data was analyzed using Up-down reader, which is an open-source program that helps for efficient determination of 50% von Frey thresholds [
31].
2.6. Pathological Analyses
Mice were euthanized at 16 weeks after surgery by cervical dislocation and decapitation. The brain was divided into hemispheres and one hemisphere (ipsilateral to injection) was placed in fresh 4% paraformaldehyde. The duodenum and colon were also placed in fresh 4% paraformaldehyde. Samples were incubated at 4°C, with rocking, for 48-72 hours. Samples were then placed in 30% sucrose for a further 48-72 hours and then briefly washed with 0.01M PBS, excess liquid dried off and then they were snap-frozen in liquid nitrogen. Samples were stored at minus 80°C until processing. Serial coronal cryosections (40μm) were taken, which were placed in cryoprotectant and stored at minus 20°C until processing.
2.6.1. Histochemistry
One section per mouse, approximately AP +0.4 was stained for cresyl violet to confirm microinjection location as per [
23]. Photomicrographs were taken using a stereomicroscope (Zeiss Axio imager) at x17 magnification to confirm appropriate needle placement.
2.6.2. Immunohistochemistry and Stereology
In order to eliminate any bias, a section from the first six sections containing the SNpc was chosen at random (using the Excel Rand function). This section, and every 6th therafter (thus, spaced at 240-micron distances), were used. The series of sections were washed and then endogenous peroxidases inactivated (1% H2O2 in 0.5% Triton X-100 in PBS; 20 min). Sections were then blocked 5% goat serum (Jackson laboratories) in 0.5% TX-100 in 0.01M PBS for 30 minutes and incubated in primary antibody overnight (anti-tyrosine hydroxylase (TH) antibody (Millipore Cat# AB152, RRID:AB_390204; 1:1000 in block)). On the following day, sections were washed and incubated in secondary antibody (goat anti-rabbit biotinylated antibody; Jackson ImmunoResearch; 1:200 in blocking solution) for 2 hours. Following washing, sections were incubated in Vectastain Elite ABC Reagent in PBS containing 0.2% Triton X-100 for 2 hours. Sections were washed and then developed in 0.03% 3-3-diaminobenzidine tetrahydrochloride containing 0.0006% H2O2 in 0.05 M Tris buffer, pH 7.6. Development was monitored carefully and then sections washed in TB to stop the reaction (approx. time to development: 16 minutes). Sections were mounted onto gelatin-coated slides, dehydrated and defatted and then cover slipped. Control sections that were run in parallel and not exposed to primary antibody showed no staining.
For stereology (3D estimation of SNpc TH-positive neurons): The SNpc was outlined in each of the sections using StereoInvestigator V5.00 on a Zeiss Z1 microscope at x5 magnification. Briefly, the anterior to posterior extent of the SN was identified based on a standard mouse brain atlas (
https://mouse.brain-map.org/static/atlas) using the following landmarks: rostral aspect of the SNpc began with the first TH-positive cells near the caudal end of the subthalamic nucleus; caudal SNpc ended where the retrorubral field became visible. The optical fractionator method (Stereoinvestigator V5.00) was used to count dopaminergic neurons. Cell counts were performed at 60x using a 1.4 NA lens and 1.4 NA oil condenser, with a DVC real-time digital camera. Neurons were defined as having large, strongly TH-positive soma. The counting frames were distributed using a sampling grid of 150 × 150 µm. Counting frame sizes were 60 x 60 µm. Gunderson coefficients of error were always less than 0.1. Damage to two sections in one rotenone-treated mouse and the loss of a stereological series in one control-treated mouse resulted in group sizes of 9 and 11, respectively, for stereological data.
2.6.3. Whole-Slide Scanning and 2D Estimation of SNpc TH-Positive Neurons
Slides were scanned using 3DHistech Pannoramic Flash III 250 scanner at a 20 × magnification and analysed using SlideViewer (V2.7). The total number of TH-positive neurons in SNpc were counted per section, and this total was multilied by 6 (stereological series was 1:6). These total number of each sections containing SNpc through the stereological series was then added together to generate the mouse’ total number of SNpc neurons.
2.6.4. Immunofluorescence
GFAP: Two sections from duodenum and colon were taken from each mouse and immunostained using anti-GFAP (1:500, Atlas Antibodies Cat# HPA056030, RRID:AB_2683015). Two sections from brain (AP: -3.4 mm) were also stained in the same manner. These sections were adjacent to sections used for stereology, to enable selection of SNpc and SNpr. Group sizes (N=9 control, N=9 rotenone) were smaller for pons GFAP as it was not present in some sections.
LAMP1: Three sections from colon were taken from each mouse and stained using anti-LAMP1 (1:1500, DSHB Antibodies Cat# 1D4B-c, RRID:AB_2134500).
PGP9.5: Three sections from colon were taken from each mouse and stained using anti-PGP9.5 (1:200, UCHL1 Antibodies Cat# aa171-220, RRID: AB_2210511).
Striatal TH: Three sections from each mouse approximately AP + 0.8mm were stained using anti-TH (Millipore Cat# AB152, RRID:AB_390204; 1:500 in block).
For immunofluorescence, staining protocols were as per [
23]. Control sections that were not exposed to primary antibody were always run in parallel, and no specific staining was observed in these sections. Counterstaining for immunofluorescence was performed using 1 µg/ml Hoechst-34580 dye (Sigma Aldrich) for 10 minutes, followed by washing in TB and then sections were mounted onto gelatin-coated slides.
2.7. Image Analyses for Immunofluorescence
For gut GFAP: Z-stacks through the depth of the area of interest were taken using an LSM780 confocal (x20; 708.49x 708.49 µm; 1.38 x 1.38 x 2.65 μm/pixel; frame size: 512 x 512 μm). Images were batch-processed in ImageJ. Briefly, channels were split and the GFAP channel was made into a maximum-intensity projection, converted to 8-bit and then auto-thresholded. The longitudinal and circular muscle was outlined, and the percent area containing signal per image quantified.
For colon LAMP1: Z-stacks through the depth of the area of interest were taken using an LSM780 confocal (x40; 212.55 x 212.55 µm; 0.42 x 0.42 x 0.54 μm/pixel; frame size: 512 x 512 μm). Images were batch-processed in ImageJ as above. The outer muscle layers (longitudinal and circular) were scored manually by a blinded individual for extent of staining (0, +, ++).
For colon PGP9.5: Photomicrographs of colon were taken at x10 using cellSens Entry, V2.2 software (Olympus Life Science, Center Valley, Pennsylvania) on an Olympus IX70 microscope. To ensure consistency, all pictures were taken using the same settings, having calibrated brightness across the field of view and black-balanced the camera. For analysis, images were converted to grayscale. The mean intensity of staining within outlined ROIs of the longitudinal and circular muscle was quantified.
For brain GFAP: Photomicrographs containing of pons, SNpc and SNpr were taken using an LSM780 confocal (x10; 1024 x 1024 µm; 1.38 x 1.38 μm/pixel). Images were batch-processed in ImageJ; briefly, channels were split and the GFAP channel made into maximum-intensity projections, converted to 8-bit, then auto-thresholded. Based upon the ROI of SNpc from the adjacent TH-stained section used for stereology (see above), an ROI was drawn carefully around the SNpc and SNpr of GFAP-stained sections, and the percent area containing staining was quantified.
For TH immunofluorescence of striatum, photomicrographs were taken at x4 using cellSens Entry, V2.2 software (Olympus Life Science, Center Valley, Pennsylvania) on an Olympus IX70 microscope. To ensure consistency, all pictures were taken using the same settings, having calibrated brightness across the field of view and black-balanced the camera. Photomicrographs of sections were stitched together (Autostitch), and integrated density of striatal fluorescence was analysed using ImageJ [
32].
2.8. Statistics
All behavioral tests, pathological tests and analyses were conducted by an experimenter blinded to treatment. The null hypothesis that there was no significant difference between groups was rejected if p < 0.05. To compare one factor between two separate groups, unpaired T-tests were used, or Mann Whitney U tests, if data were non-parametric. Where one factor was compared over time within a particular group, 1-way ANOVAs were used, followed by appropriate post-hoc testing. In the case of two factors, two-way ANOVAs followed by Šídák’s multiple comparisons tests were used. Three-way ANOVAs, followed by appropriate post-hoc tests, were used where there were three factors (e.g., temperature zone, treatment, and time post microinjections for hot-and-cold exploration test). GraphPad Prism V9.3.1 was used for the majority of statistical analyses. Sample-size calculations for repeated tests were performed using GLIMMPSE software [
33]. Sample-size calculations for single-timepoint testing were performed using Clincalc (
https://clincalc.com/) and G*Power [
34].
In ClinCalc, the study group design was selected as “Two independent study groups”, the primary endpoint was continuous, alpha was set to 0.05 and power to 80%. For G*Power calculations, the test family was t-tests, which was set to independent and two-tailed, and power and sample sizes were calculated using an allocation ratio 1:1. Our trial adheres to ARRIVE 2.0 guidelines [
22]. Data are available within File S1.
4. Discussion
PD has historically been characterised as a neurodegenerative disorder affecting primarily the brain [
3,
4]. Given that central models of PD are primarily used for assessment of therapeutics in PD [
21], and that previous data suggested non-motor deficits following central administration of rotenone [
20], in this study, we sought to comprehensively analyse sensory behaviour following intrastriatal administration of rotenone.
Several of the motor symptoms of PD are caused by the loss of DA-containing neurons projecting from the
Substantia nigra to the dorsal striatum and patients typically have almost 70% TH-positive neuronal loss in SNpc [
49]. Thus, most animal models where a neurotoxin induces a strong and rapid cell loss in the substantia nigra pars compacta (SNpc) elicit motor symptoms [
12]. Here, all animals injected with rotenone were effectively lesioned, as they showed a 50% loss in DA neurons in the SNpc and a 49% loss in TH-positive terminals in striatum, similar to other published findings [
12], and lesioned mice showed elevated GFAP-labelled astrocytes in SN.
We counted TH-positive neurons in SNpc in 3D, using an optical fractionator, and numbers in normal animals and in lesioned animals matched well with previous studies [
19,
20,
35]. Interestingly, we also quantified SNpc TH-positive neurons from whole-slide scans, with simple 2D counting. We did not use a correction, for example the Abercombie correction [
50], as this is appropriate if successive samples are counted because it is used to compensate for lost caps and our sections were a 1:6 stereological series. The number of SNpc TH-positive neurons was lower, and the relative difference between groups declined. Thus, we note that 2D-based counts are less sensitive than traditional 3D optical fractionator-based counts [
36].
Intrastriatal rotenone caused robust motor impairments in hindlimb stepping and motor coordination and balance on the accelerating rotarod paradigm. These behavioural tests are legimate and sensitive tests of striatal integrity and performance in hindlimb stepping is improved with levodopa [
25,
26]. However, we note that the rotarod paradigm showed large variability and requires large group sizes. We detected minor changes in gait, which is reminiscent of the relative lack of senstivity of this task when SNpc TH-positive neuronal loss is less than 80-90% [
39,
40]. Forelimb grip strength was unaffected in the rotenone-treated group. Interestingly, all-four-paw grip strength tended to be lower in the rotenone-treated group, but was inconsistently statistically significant. This again indicates a lack of sensitivity of this test. PD patients show a decline in hand-grip strength with increasing UPDRS score or Hoehn and Yahr scale, which is independent of years since diagnosis [
51,
52] but again, almost 70% loss in TH-positive neurons in SNpc is noted in human tissue from PD patients (see above)[
49]. No change was noted in spontaneous activity, again showing this task’s relative lack of sensitivity to 50% loss in TH-positive SNpc neurons. Thus, our rotenone-treated mice were parkinsonian but may not have been as impaired as patients diagnosed with PD because their loss in dopamine neurons was reduced compared with typical PD patients. This was a great advantage, as it enabled study of non-motor signs at early disease progression (based upon loss of dopamine neurons alone).
A well-known non-motor prodromal symptom of PD is GI dysfunction [
3,
4] with a prevalence of 70–80% and characterised by bloating and delayed gastric emptying [
53]. We examined GI motility using carmine red, a non-absorbable dye that is well-validated for whole gastrointestinal transit time in humans [
43] and rodents [
28,
44]. We never observed delayed expulsion of carmine-red-coloured faeces in our rotenone-treated mice. Further, we observed no change in GFAP expression in colon or duodenum, which is a marker of enteric glial cells that is elevated in PD [
45]. Importantly, repeated testing further increases robustness of our data, and indeed a lack of repeated testing has been identified as an issue in PD preclinical research [
21]. As stated above, we define our model as early parkinsonism because we observed 50% loss in TH-positive neurons in SNpc (patient typically show 70% loss), and we observed consistent, robust and specific motor deficits on the accelerating rotarod and in the hindlimb stepping task, only. Moreover, because expected peripheral signs (reduced GI motility) were absent, mice were parkinsonian but did not model PD.
In this traditional model of PD, our primary goal was to examine sensation of heat, cold and touch, as disorders of the skin are being increasingly recognized in PD [
5], PD patient skin shows aggregated alpha synuclein [
7], and we have shown extensive alterations in the skin transcriptome of PD patients [
54]. A recent meta-analysis revealed, in particular, increased sensitivity to cold pain in PD patients [
47]. Incorporating sensory assessments alongside traditional motor assessments can provide a more complete picture of the disease, especially as counts of intraepidermal nerve fibres decline as PD progresses [
55]. Interestingly, sensory neuronal impairment may explain, in part, some of the motor deficits in PD [
56]. Additionally, sensory deficits are important prior to disease: skin complaints rise in the years prior to diagnosis of PD [
5] and peripheral neuropathy is observed in PD patients at diagnosis [
57]. However, we did not observe any behavioural changes in heat, cold, or touch sensitivity in our mice. PD patients are much less likely to show changes in sensation in the area of the face and are more likely to show changes in upper or lower limb [
47], thus we focused on sensation in distal limbs. Again, we used statistically well-powered and well-validated behavioural tests and we tested mice repeatedly. Thus, our data are clear indications of the failure of central DA loss alone to replicate PD although it does mirror parkinsonism (motor phenotypes). These data are reminiscent of data from primates showing that MPTP, which primarily kills DA neurons in brain, fails to cause extensive changes in gut motility or may even increase it [
58]. Additionally, previous sensory testing revealed increased sensitivity to heat or pin-point pressure in rats lesioned with 6-OHDA [
59,
60], another traditional method to induce dopaminergic cell loss in the SNpc. However, PD patients show increased sensitivity to cold pain most consistently [
47].
Our data show that it is essential to take a comprehensive approach that considers the complexity of PD rather than dopaminergic neuronal loss. A recent reviewed showed that the most typical models used to test potential disease modifying therapeutics were toxic models (MPTP or 6-OHDA)[
21]. Moreover, sample size calculations were “uniformly absent” from the papers they reviewed. A lack of sample size calculations also occurs in preclinical Alzheimer’s disease research [
61] and in preclinical research on traumatic brain injury [
62]; however, for example, we recently published sample sizes and expected power for many outcome measures for preclinical Alzheimer’s disease research [
23] and we present further calculations in this paper. Certainly, MPTP and 6-OHDA models will develop central dopaminergic cell death and associated motor disorders, but our data reiterate that this is not sufficient to model the disease. Moreover, our data suggest that non-motor signs may not respond to levodopa, which has been shown to be the case in human PD patients [
3], and indeed, high-dose levodopa may even contribute to peripheral neuropathy in PD [
63,
64].
In conclusion, our data show the importance of repeated testing in preclinical studies to reduce variability and group sizes for several behavioural outcome measures. Our behavioural testing used well-powered and well-validated tests that were suited to rodents but that have high translational relevance to humans [
62,
65]. Critically, and most importantly, our data show no peripheral behavioural effects following central administration of rotenone. Thus, central modelling does not reproduce expected peripheral signs of PD (gut motility) and also fails to induce sensory changes, which are increasingly recognised in PD but are little understood. Our data have important implications for current preclinical therapeutics testing in PD, which relies heavily on toxic central-based models, and further, our data suggest that more comprehensive models are required to address peripheral signs in PD, which are a priority for development.
Figure 1.
A) Mean integrated fluorescence intensity of TH in striata from control (N=12) and rotenone-treated (N=10) mice. Rotenone caused a significant reduction in striatal TH-positive terminals. ****p<0.0001. Symbols are of individual mice and horizontal bars show mean ± sem. B) Loss of dopaminergic (DAergic) neurons in SNpc in rotenone-treated (N=9) mice compared with controls (N=11). ****p<0.0001. Symbols are of individual mice and horizontal bars show mean ± sem. C) Representative photomicrograph of DAergic neurons in the SNpc of a control mouse (top) and a representative photomicrograph showing loss of DAergic neurons in a rotenone-treated mouse (bottom).
Figure 1.
A) Mean integrated fluorescence intensity of TH in striata from control (N=12) and rotenone-treated (N=10) mice. Rotenone caused a significant reduction in striatal TH-positive terminals. ****p<0.0001. Symbols are of individual mice and horizontal bars show mean ± sem. B) Loss of dopaminergic (DAergic) neurons in SNpc in rotenone-treated (N=9) mice compared with controls (N=11). ****p<0.0001. Symbols are of individual mice and horizontal bars show mean ± sem. C) Representative photomicrograph of DAergic neurons in the SNpc of a control mouse (top) and a representative photomicrograph showing loss of DAergic neurons in a rotenone-treated mouse (bottom).
Figure 2.
A) GFAP fluorescence intensity, depicted as percent area above threshold, in SNpc+SNpr or in pons. Post hoc test: * p<0.05. Symbols are of individual mice for SNpc (N=11 control, N=10 rotenone) or pons (N=9 each). Horizontal bars show mean ± sem. SNpc, Substantia nigra pars compacta; SNpr, Substantia nigra pars reticulata. B) Representative image of GFAP expression at x20, in SN in a control-treated mouse. Outline shows SNpc, for reference, based upon an adjacent section stained for TH and used for stereology. C) Representative image at x20, showing an increase in GFAP expression in SN in a rotenone-treated mouse. Outline shows SNpc, for reference, based upon an adjacent section stained for TH and used for stereology. Scalebar in upper right= 50μm, for both photomicrographs.
Figure 2.
A) GFAP fluorescence intensity, depicted as percent area above threshold, in SNpc+SNpr or in pons. Post hoc test: * p<0.05. Symbols are of individual mice for SNpc (N=11 control, N=10 rotenone) or pons (N=9 each). Horizontal bars show mean ± sem. SNpc, Substantia nigra pars compacta; SNpr, Substantia nigra pars reticulata. B) Representative image of GFAP expression at x20, in SN in a control-treated mouse. Outline shows SNpc, for reference, based upon an adjacent section stained for TH and used for stereology. C) Representative image at x20, showing an increase in GFAP expression in SN in a rotenone-treated mouse. Outline shows SNpc, for reference, based upon an adjacent section stained for TH and used for stereology. Scalebar in upper right= 50μm, for both photomicrographs.
Figure 3.
A) Body weights from control- (N=12) and rotenone-treated (N=10) mice, expressed as percent of starting weight. Asterisks indicate significant differences between control and treated groups. Post hoc tests: *p<0.05, **p<0.01. Symbols show group mean ± sem. Note that sem are obscured within symbols for many datapoints. B) Activity in a novel environment (open field activity) over a period of 1 hour in control- (N=12) and rotenone-treated (N=10) mice. No difference was detected between groups. Symbols are of individual mice and horizontal bars show group mean ± sem.
Figure 3.
A) Body weights from control- (N=12) and rotenone-treated (N=10) mice, expressed as percent of starting weight. Asterisks indicate significant differences between control and treated groups. Post hoc tests: *p<0.05, **p<0.01. Symbols show group mean ± sem. Note that sem are obscured within symbols for many datapoints. B) Activity in a novel environment (open field activity) over a period of 1 hour in control- (N=12) and rotenone-treated (N=10) mice. No difference was detected between groups. Symbols are of individual mice and horizontal bars show group mean ± sem.
Figure 4.
A) Forepaw grip strength from control- (N=12) and rotenone-treated (N=10) mice. No difference between groups was observed. Symbols show individual mice and horizontal bars show mean ± sem. B) All-four-paw grip strength from control- (N=12) and rotenone-treated (N=10) mice. Although rotenone-treated mice in general showed reduced grip strength, it was only specifically significant at 10 weeks. * p<0.05 Symbols show individual mice and horizontal bars show mean ± sem. C) Measurements of stride length of forelimbs and hindlimbs from control- (N=12) and rotenone-treated (N=10) mice. A minor deficit in forelimb stride length, only, was observed. * p<0.05. Symbols show individual mice and horizontal bars show mean ± sem. D) Measurements of stride width of forelimbs and hindlimbs from control- (N=12) and rotenone-treated (N=10) mice. No differences between groups were observed. Symbols show individual mice and horizontal bars show mean ± sem.
Figure 4.
A) Forepaw grip strength from control- (N=12) and rotenone-treated (N=10) mice. No difference between groups was observed. Symbols show individual mice and horizontal bars show mean ± sem. B) All-four-paw grip strength from control- (N=12) and rotenone-treated (N=10) mice. Although rotenone-treated mice in general showed reduced grip strength, it was only specifically significant at 10 weeks. * p<0.05 Symbols show individual mice and horizontal bars show mean ± sem. C) Measurements of stride length of forelimbs and hindlimbs from control- (N=12) and rotenone-treated (N=10) mice. A minor deficit in forelimb stride length, only, was observed. * p<0.05. Symbols show individual mice and horizontal bars show mean ± sem. D) Measurements of stride width of forelimbs and hindlimbs from control- (N=12) and rotenone-treated (N=10) mice. No differences between groups were observed. Symbols show individual mice and horizontal bars show mean ± sem.
Figure 5.
A) Rotarod performance of control (N=12) and rotenone-treated (N=10) mice. Rotenone-treated mice showed a robust decline in performance. Symbols show group mean ± sem. B) Number of continuous hindlimb steps in control- (N=12) and rotenone-treated (N=10) mice. A significant reduction in number of continuous hindlimb steps in rotenone-treated mice was observed. Asterisks indicate significant differences compared with baseline, *p<0.05, **p<0.01, and ****p<0.0001. Symbols show group mean ± sem.
Figure 5.
A) Rotarod performance of control (N=12) and rotenone-treated (N=10) mice. Rotenone-treated mice showed a robust decline in performance. Symbols show group mean ± sem. B) Number of continuous hindlimb steps in control- (N=12) and rotenone-treated (N=10) mice. A significant reduction in number of continuous hindlimb steps in rotenone-treated mice was observed. Asterisks indicate significant differences compared with baseline, *p<0.05, **p<0.01, and ****p<0.0001. Symbols show group mean ± sem.
Figure 6.
A) Latency to expel red faecal pellets following administration of the non-absorbable dye carmine red in control- (N=12) and rotenone-treated (N=10) mice, i.e., whole-gut motility. No difference in latency was noted in repeatedly tested rotenone-treated mice compared with control-treated mice. Symbols are of group mean ± sem. B) GFAP-positive staining, depicted as percent above background, in duodenum and colon from control- (N=12) and rotenone-treated (N=10) mice. No change was observed between groups. Symbols are of individual mice and horizontal bars show group mean ± sem. Photomicrographs depict exemplar images from colon section, control (top) and rotenone (bottom, scalebar = 50μm, for both photomicrographs). Dotted lines depict outer muscle layers (longitudinal + circular). C) Mean integrated fluorescence intensity of PGP 9.5, a pan-neuronal marker that was used to detect enteric ganglia in longitudinal and circular muscle layers of colon from control (N=12) and treated (N=10) mice. No change was observed between groups. Symbols are of individual mice and horizontal bars show group mean ± sem. Photomicrographs depict exemplar images from colon section, control (top) and rotenone (bottom; bottom, scalebar = 100μm, for both photomicrographs). Dotted lines depict outer muscle layers (longitudinal + circular). D) LAMP1-positive staining was scored on a criteria of 0, + or ++ in outer longitudinal and circular muscle layers of colon from control- (N=12) and rotenone-treated (N=10) mice. No change was observed between groups. Symbols are of individual mice and horizontal bars show group mean ± sem. Photomicrographs depict exemplar images from colon, control (top) and rotenone (bottom, scale bar = 100μm, for both photomicrographs). Dotted lines depict outer muscle layers (longitudinal + circular).
Figure 6.
A) Latency to expel red faecal pellets following administration of the non-absorbable dye carmine red in control- (N=12) and rotenone-treated (N=10) mice, i.e., whole-gut motility. No difference in latency was noted in repeatedly tested rotenone-treated mice compared with control-treated mice. Symbols are of group mean ± sem. B) GFAP-positive staining, depicted as percent above background, in duodenum and colon from control- (N=12) and rotenone-treated (N=10) mice. No change was observed between groups. Symbols are of individual mice and horizontal bars show group mean ± sem. Photomicrographs depict exemplar images from colon section, control (top) and rotenone (bottom, scalebar = 50μm, for both photomicrographs). Dotted lines depict outer muscle layers (longitudinal + circular). C) Mean integrated fluorescence intensity of PGP 9.5, a pan-neuronal marker that was used to detect enteric ganglia in longitudinal and circular muscle layers of colon from control (N=12) and treated (N=10) mice. No change was observed between groups. Symbols are of individual mice and horizontal bars show group mean ± sem. Photomicrographs depict exemplar images from colon section, control (top) and rotenone (bottom; bottom, scalebar = 100μm, for both photomicrographs). Dotted lines depict outer muscle layers (longitudinal + circular). D) LAMP1-positive staining was scored on a criteria of 0, + or ++ in outer longitudinal and circular muscle layers of colon from control- (N=12) and rotenone-treated (N=10) mice. No change was observed between groups. Symbols are of individual mice and horizontal bars show group mean ± sem. Photomicrographs depict exemplar images from colon, control (top) and rotenone (bottom, scale bar = 100μm, for both photomicrographs). Dotted lines depict outer muscle layers (longitudinal + circular).
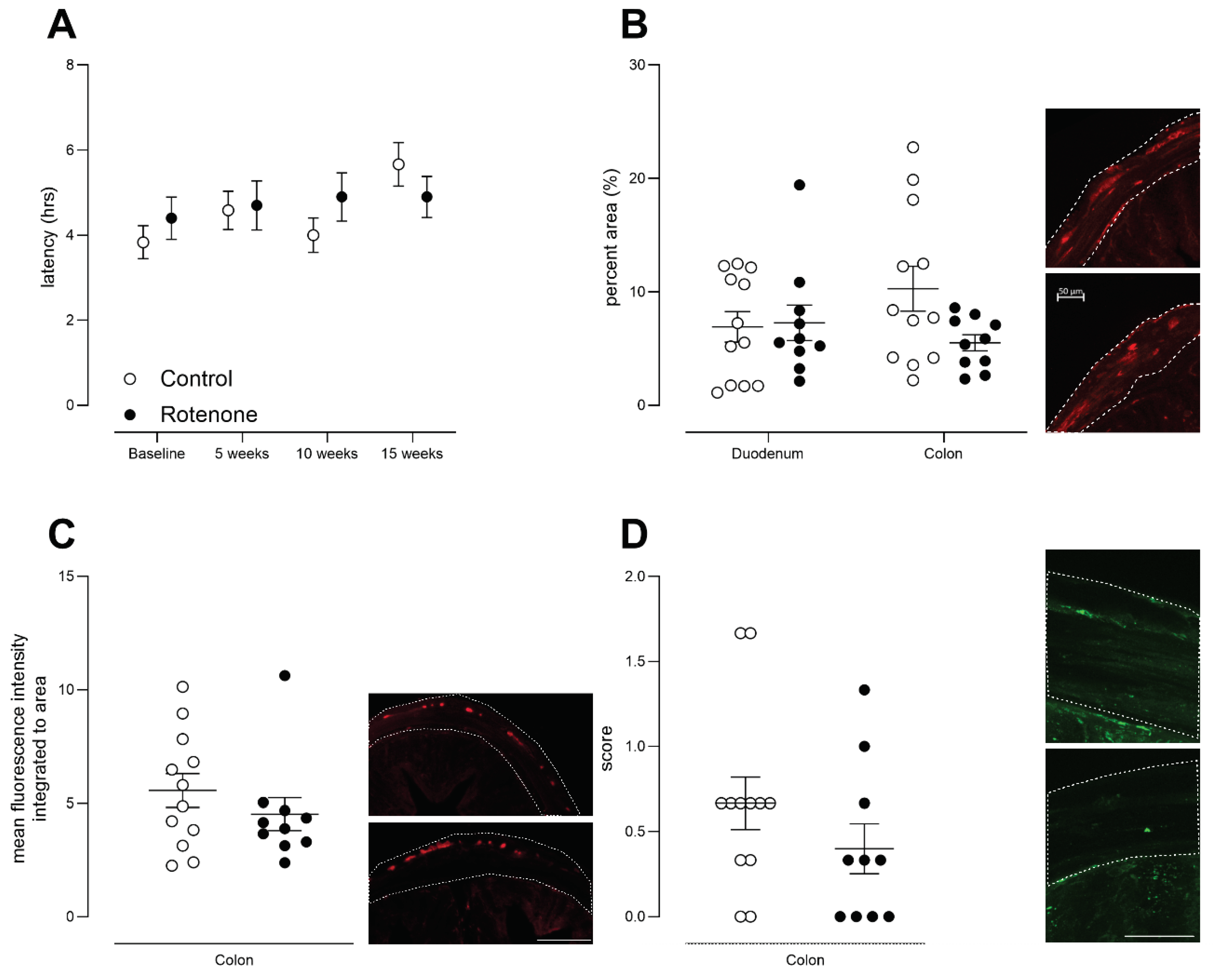
Figure 7.
A) The latency to withdraw hind-paw from heat in control- (N=12) and rotenone-treated (N=10) mice. Symbols are of individual mice and horizontal bars show group mean ± sem. B) The latency to withdraw tail from heat in control- (N=12) and rotenone-treated (N=10) mice. Symbols are of individual mice and horizontal bars show group mean ± sem. C) The latency to withdraw hind-paw from cold in control- (N=12) and rotenone-treated (N=10) mice. Although this model (intrastriatal rotenone) has been shown to result in bilateral SNpc TH-positive neuronal loss [
20], we tested each hindlimb in the cold-plantar test but this enhanced outcome also failed to reveal any deficit in sensation. Symbols are of individual mice and horizontal bars show group mean ± sem.
Figure 7.
A) The latency to withdraw hind-paw from heat in control- (N=12) and rotenone-treated (N=10) mice. Symbols are of individual mice and horizontal bars show group mean ± sem. B) The latency to withdraw tail from heat in control- (N=12) and rotenone-treated (N=10) mice. Symbols are of individual mice and horizontal bars show group mean ± sem. C) The latency to withdraw hind-paw from cold in control- (N=12) and rotenone-treated (N=10) mice. Although this model (intrastriatal rotenone) has been shown to result in bilateral SNpc TH-positive neuronal loss [
20], we tested each hindlimb in the cold-plantar test but this enhanced outcome also failed to reveal any deficit in sensation. Symbols are of individual mice and horizontal bars show group mean ± sem.
Figure 8.
The 50% withdrawal threshold in control- (N=12) and rotenone-treated (N=10) mice. No change in sensation of punctate mechanical stimulus was noted in rotenone-treated mice compared with controls. Symbols are of individual mice and horizontal bars show group mean ± sem.
Figure 8.
The 50% withdrawal threshold in control- (N=12) and rotenone-treated (N=10) mice. No change in sensation of punctate mechanical stimulus was noted in rotenone-treated mice compared with controls. Symbols are of individual mice and horizontal bars show group mean ± sem.
Figure 9.
Spontaneous preference for hot or cold environments was measured in control- (N=12) and rotenone-treated (N=10) mice over a 30-minute period. We did not detect any difference in preference for any particular temperature zone in rotenone-treated mice compared with controls. Symbols are of individual mice, and horizontal bars show group mean ± sem.
Figure 9.
Spontaneous preference for hot or cold environments was measured in control- (N=12) and rotenone-treated (N=10) mice over a 30-minute period. We did not detect any difference in preference for any particular temperature zone in rotenone-treated mice compared with controls. Symbols are of individual mice, and horizontal bars show group mean ± sem.
Table 1.
Group sizes required to detect a 50% or 30% difference from control, based upon single-timepoint testing or repeated testing.
Table 1.
Group sizes required to detect a 50% or 30% difference from control, based upon single-timepoint testing or repeated testing.
Behvioral outcome measure |
Group size required to see a 50% change from control α = 0.05 80% power |
Group size required to see a 30% change from control α = 0.05 80% power |
|
Single-timepoint testing |
Repeated Testing |
Single-timepoint testing |
Repeated Testing |
|
ClinCalc |
G-Power |
Glimmpse |
ClinCalc |
G-Power |
Glimmpse |
Open field distance |
6 |
7 |
101
|
15 |
17 |
241
|
Grip strength (all four paws) |
02
|
2 |
31
|
1 |
3 |
41
|
Grip strength (fore paws) |
5 |
6 |
31
|
13 |
14 |
51
|
Hindlimb stepping |
2 |
4 |
91
|
5 |
7 |
231
|
Rotarod |
6 |
8 |
233
|
18 |
19 |
623
|
Tail flick |
6 |
7 |
31
|
16 |
17 |
41
|
Hargreaves |
3 |
5 |
91
|
9 |
11 |
231
|
von Frey |
14 |
16 |
91
|
39 |
41 |
231
|
Cold plantar |
6 |
7 |
94
|
16 |
17 |
214
|
Hot & and cold preference |
11 |
13 |
75
|
30 |
32 |
165
|
Gut motility |
4 |
6 |
71
|
11 |
12 |
181
|