1. Introduction
Cyclin-dependent kinases (CDKs) are serine-threonine kinases that require binding with regulatory proteins called cyclin to be active. CDKs are the main regulators of the cell cycle and gene transcription. The human proteome contains 20 CDKs and 29 cyclins. CDK1 to CDK6 are involved in cell cycle regulation, while CDK7, CDK8, CDK9, CDK11, and CDK20 are primarily involved in transcriptional regulation. More particularly, CDK7, CDK8 and CDK9 control the activity of RNA polymerase II in humans by the phosphorylation of its C-terminus domain, which catalyzes the synthesis of all mRNA precursors [
1]. The inhibition of CDK activity by small molecules for the treatment of cancer has been extensively studied [
2]. Several CDK inhibitors have undergone clinical trials and in February 2015 palbociclib, a CDK4/6 inhibitor, was the first approved by the FDA [
3].
CDK8 is a target of interest that has recently attracted considerable attention after the publication of numerous genetic and biochemical studies highlighting its many key roles in oncogenesis [
4,
5]. Among its various cellular functions, the most notable is its involvement in regulating transcription trough diverse mechanisms. CDK8 is a part of the mediator complex, which is a large multisubunit protein complex that is central to the regulation of transcription in eukaryotes [
6]. The main function of the mediator complex is to transmit regulatory signals from DNA-bound transcription factors to the RNA polymerase II (RNAPII). The complex CDK8-Cyclin C (CDK8-CycC) associates with MED12 and MED13 to form the CDK8 module, a sub-module of the mediator complex [
7,
8,
9]. In humans, it has been demonstrated in vitro that the CDK8 module inhibits the initiation of transcription by deactivating CDK7, which can no longer phosphorylate the carboxy-terminal domain of RNAPII, thereby blocking the transcription [
10]. On the other hand, contrary to this transcriptional repression role, it has been observed
in vivo, a positive regulatory role for CDK8 via the recruitment of SEC (Super Elongation Complex). In fact, the interaction of the mediator complex with SEC facilitates the elongation and release of certain genes [
11,
12]. In particular, CDK8-mediated activation of the Wnt-β-catenin signaling pathway [
13] and of the transcription of estrogen-inducible genes [
14] contribute, respectively, to oncogenesis in colorectal and mammary tumors, making CDK8 an oncogene of interest.
Since Schneider et al. published in 2011 the first crystallographic structure of human CDK8-CycC complexed with sorafenib (PDB id.: 3RGF) [
15], a total of 31 experimental structures are currently available. All of these crystal structures present 10 to 20 missing residues within a region that lies outside the active-site cleft called the activation loop. This motif has a central role in regulating the activity of protein kinase by generally adopting a
DFG-in conformation in the active form and a
DFG-out conformation in the inactive form [
16]. In that connection, the first computational study on human CDK8 (with PDB id.: 3RGF) aims at providing insights into two point mutations within the activation loop through 50 ns of all-atom conventional molecular dynamics (cMD) simulation in implicit solvent [
17]. Moreover, the theoretical binding free energy between CDK8 and CycC was also determined using MM-PBSA and MM-GBSA on the basis of 2 ns of all-atom cMD simulation in explicit solvent. However, in in silico structural studies, a particular attention should be paid to the building of a relevant model of the protein, especially in this study [
17] where the object of the investigation, the activation loop, is missing and has to be reconstructed. Surprisingly, the authors used a template where the activation loop is in
DFG-in conformation to model the activation loop of 3RGF (PDB id.), which is in
DFG-out conformation. Cholko et al. studied twelve CDK8-CycC systems using 500 ns all-atom cMD simulations in explicit solvent with the aim of elucidating the system motions and the structural determinants that affect protein-ligand interactions [
18]. They find that the CycC is important in providing proper interactions for ligand binding whereas the highly flexible activation loop has a little effect. Furthermore, they employed MM-PBSA analysis to characterize protein-ligand interactions from an energetically point of view and discuss the major driving force of protein-ligand binding.
In this study, we investigated the effect of CycC on the structure and dynamics of CDK8 on the one hand, and on the other hand, the structural molecular basis of the protein-protein interaction between the two partners. Indeed, the presence of CycC in the CDK8-CycC complex seems to play a more complex role than for other members of the CDK family [
19,
20]. CDKs are generally activated in two steps: 1) the binding of the cyclin (Cyc) to CDK and 2) the phosphorylation of a threonine residue in the CDK activation loop (T160 in human CDK2). The binding of the Cyc to CDK induces a conformational change of the αC-helix, which adopts a
αC-helix in conformation (shift toward the binding site) from an
αC-helix out conformation. The phosphorylated threonine on the activation loop serves as an anchor for adjusting the orientation of three conserved arginine residues, inducing a conformational change in the activation loop that shifts from a
DFG-out to a
DFG-in conformation [
21]. In CDK8 the phosphorylation step has not been observed and is not required to its activation [
22,
23]. Moreover, the first published crystallographic structures of human CDK8-CycC [
15,
24] and also a more recent one [
25] display a surprising conformation corresponding somehow to the “intermediate state of the activation mechanism”. Indeed, the αC-helix is in
αC-helix in conformation, which is expected since CycC is bound to CDK8 in agreement with the activation mechanism. However, the phosphorylation step did not occur due to the lack of the conserved threonine in the CDK8 sequence [
22], leading to keeping a
DMG-out conformation (in CDK8, a DMG motif replace the well-known DFG motif of protein kinases) for the activation loop. All of these structures are co-crystallized with an inhibitor which is told to be responsible of the conformational change from
DMG-in to
DMG-out conformation. Protein kinase inhibitors are classified based on their binding to their receptor [
26]. Type I inhibitors bind to the ATP binding site, type II inhibitors extend from the ATP binding site into a neighboring pocket, the allosteric pocket (also called “hydrophobic pocket”) which is only accessible by the rearrangement of the DFG motif from the
DFG-in to the
DFG-out conformation. The type III inhibitors bind only to the allosteric pocket. All co-crystallized inhibitors of CDK8 belong to the type II or type III class of protein kinase inhibitors. As far as we know, CDK8 is the only CDK family member for which the following structure is obtained experimentally: a
DFG-out conformation (
DMG-out in CDK8) while being associated with CycC. All CDK structures complexed with Cyc are usually in
DFG-in conformation in accordance with its activation mechanism. Alexander et al. try to reproduce this particular conformation with the complex CDK2-CycB. They incubate the CDK2-CycB complex with a type II inhibitor and also observe a
DFG-out conformation. However, they find that binding of a type II inhibitor to CDK2-CycB results in the dissociation of cyclin B from CDK2 in a competitive manner [
27]. All those observations raise the question about the role of CycC in the complex CDK8-CycC in the inactive conformation (
DMG-out). Particularly, it is interesting to investigate whether the CycC has an impact on the structure and dynamics of CDK8. In addition, this impact is the same in active (
DMG-in) and inactive (
DMG-out) conformation and in the presence and absence of the ligand has to be explored. Furthermore, in view of this unique feature of CDK8 to bind the CycC in both conformations, it is relevant to study the interaction between CDK8 and CycC in order to decipher the interaction molecular basis and to highlight possible important CDK8-specific interaction hotspots. We may notice that the particular behavior of the CycC among the cyclin family had already been raised before. This led Barette et al. [
28] to manage mutagenesis experiments that highlighted a double-point mutation of R65A/E66A in CDK8 that greatly affects its capacity to bind to CycC. This effect was partly explained by the X-ray structure which shows the contacts between Met61 and Arg65 in the human CDK8 and CycC.
Through MD simulations and binding free energy calculations, we found that CycC has a stabilizing effect on CDK8, and the importance for CDK8 to maintain a proper conformation in the active and inactive form of CDK8-CycC. The per residue free energy decomposition method enabled to characterize the CDK8-CycC binding surface, to identify the important residues and to obtain their energy contributions. We found that CDK8-CycC presents specific interaction hotspots within its interaction surface compared to other human CDK/Cyc pairs. Targeting these specific interaction hotspots could be a promising approach in terms of specificity, to effectively disrupt the interaction between CDK8 and CycC and thus, to interfere with the function of CDK8 as an oncogene. The simulation of the conformational transition from the inactive to the active form of CDK8-CycC through TMD simulation, suggests another mechanism that could substitute the missing phosphorylation step in the activation mechanism of CDK8. In a more general view, these results point the importance of keeping the CycC in computational studies when studying the human CDK8 protein in both the active and the inactive form.
4. Conclusion
Theoretical studies were conducted on the human CDK8-CycC complex in order to provide more understanding about the binding of CycC to CDK8 that is an important target in cancer therapy. We first investigated the role of CycC on the structure and dynamics of CDK8. We found that the CycC is crucial for maintaining a proper structure and dynamics of CDK8 in both the active (
DMG-in) and inactive (
DMG-out) form of the complex. Unlike CDK2, where the binding of a type II inhibitor to CDK2-CycB results in the dissociation of CycB from CDK2 in a competitive manner [
27], Schneider
et al. have shown that the binding of a type II inhibitor to CDK8-CycC does not dissociate CycC [
24]. Our findings converge to this result since the presence of a type II inhibitor does not affect the stabilizing effect of the CycC toward CDK8. The free energy values of CDK8-CycC binding calculated through the MM-GBSA method confirm these results, and show that the CycC stabilizes both CDK8 forms (active and inactive) to the same extent.
The analysis of the interaction between CDK8 and CycC, through the method of per-residue binding free energy decomposition, highlighted 26 hotspot residues uniformly distributed on the interaction surface, that strongly and favorably (ΔGtotal < -1kcal.mol-1) contribute to CDK8-CycC binding in all studied CDK8-CycC complexes. 19 of the 26 important residues belong to the conserved common interaction surface in the human CDK family. On the contrary, the remaining 7 hotspot residues are situated in two binding sites of the interaction surface that are specific to CDK8-CycC complex and involve the proline rich C-terminus segment, the CDK8 αB-helix and the N-terminus segment of CycC. These key amino acids proposed in this work are valuable information to design an inhibitor, that will effectively prevent the binding of the CycC to CDK8, which will block the activation of the complex, thereby interfering with the function of CDK8 as an oncogene. The active and the inactive forms display some differences in their CDK8-CycC binding energy contribution values. These differences might be explained by the flip of the activation loop from a DMG-out to a DMG-in conformation and the displacement of the CycC toward CDK8 in the active form.
The simulation of the conformational transition from the inactive to the active form through TMD simulation showed that this displacement of the CycC toward CDK8 occurs during the conformational change. This displacement is an important event to adjust the orientation of three conserved arginine residues (Arg65CDK8, Arg178CDK8 and Arg150CDK8), which is meditated by the Glu99CycC, thereby inducing a DMG-in conformation (active form). The active form is maintained through a hydrogen bond interaction network involving the three arginines and the Glu99CycC. In human CDK family, the three conserved arginine residues, together with a phosphorylated residue, are known to have a role in the conformational change of CDK and in the stabilization of the active form. Our TMD simulation suggests that Glu99CycC assumes the role of the missing phosphorylated residue in CDK8.
Our study provides interesting molecular insights, describing the interaction between CDK8 and CycC in terms of structure and energy. Since this interaction is essential to the activity of CDK8, the particular characteristics of this interaction and of its mechanism of activation highlighted in this study, are valuable information to design specific compounds targeting the CDK8/CycC interface. In a more general view, these results point the importance of keeping the CycC in computational studies when studying the human CDK8 protein in both the inactive and active form.
Author Contributions
Conceptualization, S.Z., P.B. and S.A.S.; methodology, S.Z., J.D. and S.A.S.; validation, S.Z., J.D. and S.A.S.; formal analysis, D.S. and S.Z.; investigation, D.S. and S.Z.; resources, P.B.; data curation, D.S. and S.Z.; writing—original draft preparation, S.Z.; writing—review and editing, S.Z., J.D., P.B. and S.A.S.; visualization, S.Z., J.D., P.B. and S.A.S.; supervision, P.B. and S.A.S.; project administration, P.B..; funding acquisition, P.B. All authors have read and agreed to the published version of the manuscript.
Figure 1.
Root mean square fluctuations (RMSF) per residue of CDK8 in presence (black) and absence (blue) of the CycC during 1 μs of simulation.
Figure 1.
Root mean square fluctuations (RMSF) per residue of CDK8 in presence (black) and absence (blue) of the CycC during 1 μs of simulation.
Figure 2.
PCA projection of the structural evolution of CDK8 in DMG-in conformation (systems 8/9, left) and DMG-out conformation (systems 1a/2a, right) in presence (black) and absence (blue) of the CycC during the MD simulations. One snapshot of a trajectory is represented by a dot in the individual map of PC1 against PC2.
Figure 2.
PCA projection of the structural evolution of CDK8 in DMG-in conformation (systems 8/9, left) and DMG-out conformation (systems 1a/2a, right) in presence (black) and absence (blue) of the CycC during the MD simulations. One snapshot of a trajectory is represented by a dot in the individual map of PC1 against PC2.
Figure 3.
Impact of the CycC on the structure of CDK8: contribution of each residue of CDK8 to PC1.Loading plot of the PCA done on the combined trajectory (with/without CycC) in DMG-in conformation system (top left) and DMG-out conformation systems (bottom left). On right representation of CDK8 in dark gray ribbon except the regions of the kinase domain containing the conserved motifs.
Figure 3.
Impact of the CycC on the structure of CDK8: contribution of each residue of CDK8 to PC1.Loading plot of the PCA done on the combined trajectory (with/without CycC) in DMG-in conformation system (top left) and DMG-out conformation systems (bottom left). On right representation of CDK8 in dark gray ribbon except the regions of the kinase domain containing the conserved motifs.
Figure 4.
CDK8-CycC binding interface: (a) Matrix of the per-residue energy contribution (ΔG without entropy). The residues are those that highly contribute to CDK8-CycC binding (absolute (ΔG) > 1 kcal.mol-1) in all studied CDK8-CycC complexes. Residues are tagged according to the secondary structure they belong to. The residues colored in pink-purple tones are those belonging to CDK8 specific binding sites. While those colored in green-yellow tones are those belonging to human CDK common binding sites; (b) CDK8/CycC structure and the binding sites at the interface CDK8-CycC. CDK8 in dark gray ribbon except the secondary structures having residues that highly contribute to CDK8-CycC binding in all studied CDK8-CycC complexes. Idem for CycC, which is colored in light gray. Besides the common binding area (represented by a dashed green box), CDK8/CycC complex forms additional contacts mediated by the CDK8 N-terminus αB-helix and the CycC N-terminus, including the HN helix (highlighted by dashed pink boxes).
Figure 4.
CDK8-CycC binding interface: (a) Matrix of the per-residue energy contribution (ΔG without entropy). The residues are those that highly contribute to CDK8-CycC binding (absolute (ΔG) > 1 kcal.mol-1) in all studied CDK8-CycC complexes. Residues are tagged according to the secondary structure they belong to. The residues colored in pink-purple tones are those belonging to CDK8 specific binding sites. While those colored in green-yellow tones are those belonging to human CDK common binding sites; (b) CDK8/CycC structure and the binding sites at the interface CDK8-CycC. CDK8 in dark gray ribbon except the secondary structures having residues that highly contribute to CDK8-CycC binding in all studied CDK8-CycC complexes. Idem for CycC, which is colored in light gray. Besides the common binding area (represented by a dashed green box), CDK8/CycC complex forms additional contacts mediated by the CDK8 N-terminus αB-helix and the CycC N-terminus, including the HN helix (highlighted by dashed pink boxes).
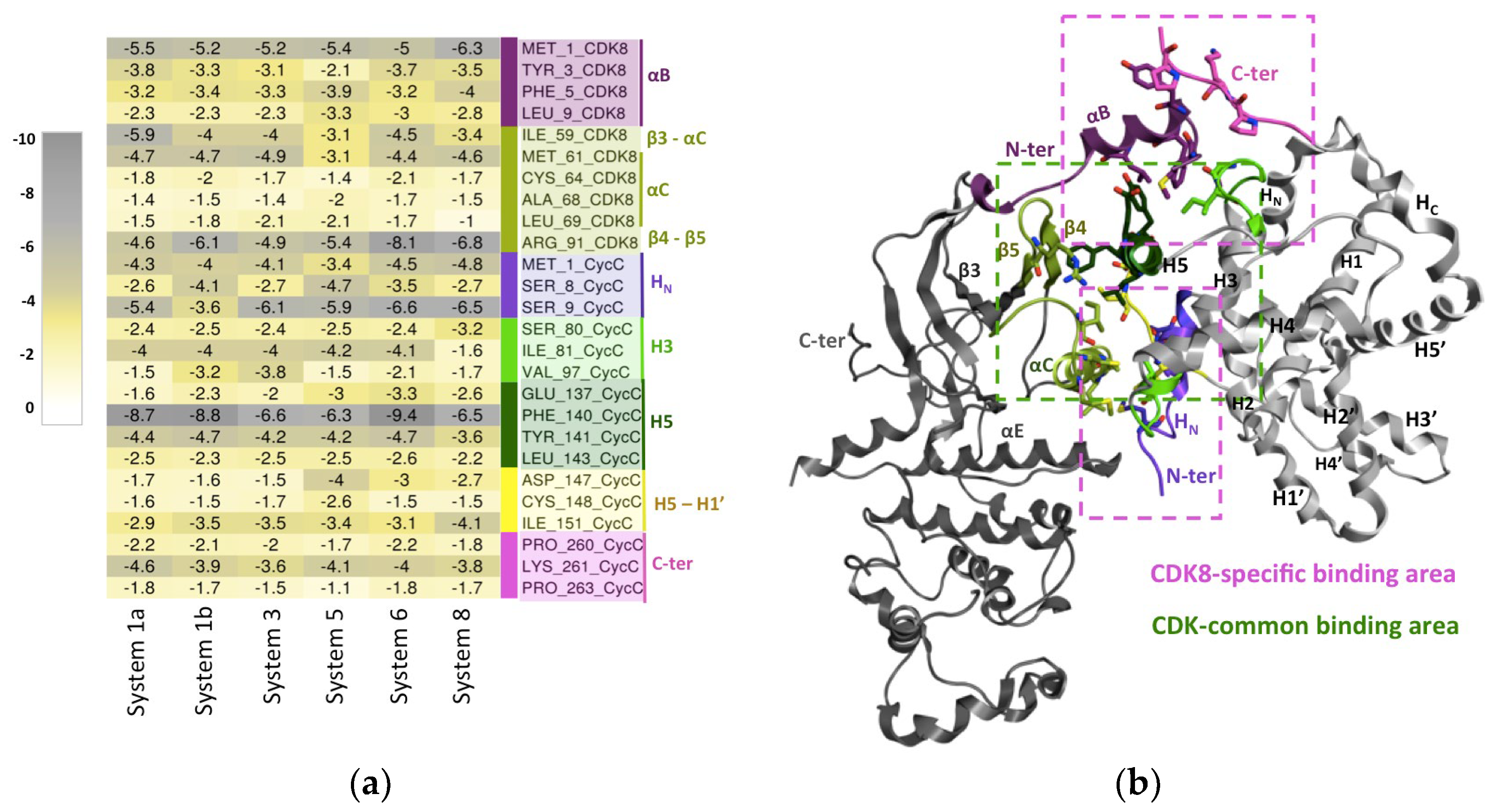
Figure 5.
Correlation plot matrix of the residues energy contributions to CDK8-CycC binding of each system. The selected residues present at least one significant energy contribution (absolute (ΔG) > 1 kcal.mol-1) in one system.
Figure 5.
Correlation plot matrix of the residues energy contributions to CDK8-CycC binding of each system. The selected residues present at least one significant energy contribution (absolute (ΔG) > 1 kcal.mol-1) in one system.
Figure 6.
Pipes and planks representation of the DMG-in and DMG-out conformations of the CDK8-CycC complexes in cartoon. CDK8 structures are colored in gray, except the activation loop. The activation loop and the CycC structures are colored in pink in the DMG-in conformation and in blue in the DMG-out conformation.
Figure 6.
Pipes and planks representation of the DMG-in and DMG-out conformations of the CDK8-CycC complexes in cartoon. CDK8 structures are colored in gray, except the activation loop. The activation loop and the CycC structures are colored in pink in the DMG-in conformation and in blue in the DMG-out conformation.
Figure 7.
Conformational transition of the CDK8 activation loop from DMG-out to DMG-in conformation: (a) plots of the measured distance between the pairs Glu99CycC/Arg178CDK8 and Glu99CycC/Lys153CDK8. The distance between Glu99CycC and Arg178CDK8 enables to monitor the transition of the activation loop over the simulation time. The distance between Glu99CycC and Lys153CDK8 enables to monitor the displacement of the CycC toward CDK8; (b) Representation of the three conserved arginines Arg65CDK8, Arg150CDK8 and Arg178CDK8 and of Glu99CycC over the simulation time course. CDK8 is represented in dark gray ribbon except the regions of the kinase domain containing the conserved motifs, particularly the activation loop is in cyan. CycC is in light gray. Arg65CDK8, Arg150CDK8, Arg178CDK8, Glu99CycC and Lys153CDK8 are represented in sticks, arginines and glutamate in light green and Lys153CDK8 in gray.
Figure 7.
Conformational transition of the CDK8 activation loop from DMG-out to DMG-in conformation: (a) plots of the measured distance between the pairs Glu99CycC/Arg178CDK8 and Glu99CycC/Lys153CDK8. The distance between Glu99CycC and Arg178CDK8 enables to monitor the transition of the activation loop over the simulation time. The distance between Glu99CycC and Lys153CDK8 enables to monitor the displacement of the CycC toward CDK8; (b) Representation of the three conserved arginines Arg65CDK8, Arg150CDK8 and Arg178CDK8 and of Glu99CycC over the simulation time course. CDK8 is represented in dark gray ribbon except the regions of the kinase domain containing the conserved motifs, particularly the activation loop is in cyan. CycC is in light gray. Arg65CDK8, Arg150CDK8, Arg178CDK8, Glu99CycC and Lys153CDK8 are represented in sticks, arginines and glutamate in light green and Lys153CDK8 in gray.
Figure 8.
Ribbon representation of the CDK8-CycC complex. Cyclin C (CycC, PDB id.: 4F6U) is colored in light grey and CDK8 in dark gray, except the conserved motifs of the kinase domain. Among these motifs, the activation loop in DMG-out conformation (inactive form) is colored in cyan (PDB id.: 4F6U) and that in DMG-in conformation (active form) in dark blue (PDB id.: 4F7S).
Figure 8.
Ribbon representation of the CDK8-CycC complex. Cyclin C (CycC, PDB id.: 4F6U) is colored in light grey and CDK8 in dark gray, except the conserved motifs of the kinase domain. Among these motifs, the activation loop in DMG-out conformation (inactive form) is colored in cyan (PDB id.: 4F6U) and that in DMG-in conformation (active form) in dark blue (PDB id.: 4F7S).
Table 1.
Comparison of the average RMSD calculated on the heavy atoms of CDK8 during 1 μs simulation for the systems with and without CycC.
Table 1.
Comparison of the average RMSD calculated on the heavy atoms of CDK8 during 1 μs simulation for the systems with and without CycC.
System id. With Cyclin C |
Average RMSD (Å) (± Standard deviation) |
System id. Without Cyclin C |
Average RMSD (Å) (± Standard deviation) |
1a |
3.6 ± 0.2 |
2a |
5.5 ± 1 |
1b |
3.9 ± 0.3 |
2b |
5.5 ± 0.3 |
3 |
3.8 ± 0.3 |
4 |
5.4 ± 0.9 |
6 |
3.2 ± 0.2 |
7 |
5 ± 0.6 |
8 |
3.4 ± 0.3 |
9 |
3.7 ± 0.4 |
Table 2.
The binding free energy and energy components of the CDK8-CycC complex calculated with MM-GBSA method and averaged on the simulations. All the energies are reported in kcal.mol-1, with their corresponding standard errors. ΔEeel and ΔEVDW are respectively electrostatic and van der Waals contributions in gas phase. ΔEGB and ΔEnp are respectively electrostatic and non-polar contributions in solvation phase. ΔGtotal is the total binding free energy without considering the entropic term.
Table 2.
The binding free energy and energy components of the CDK8-CycC complex calculated with MM-GBSA method and averaged on the simulations. All the energies are reported in kcal.mol-1, with their corresponding standard errors. ΔEeel and ΔEVDW are respectively electrostatic and van der Waals contributions in gas phase. ΔEGB and ΔEnp are respectively electrostatic and non-polar contributions in solvation phase. ΔGtotal is the total binding free energy without considering the entropic term.
Systems PDB id. Conformation
|
System 1a (4F6U) DMG-out
|
System 1b (4F6U-replica) DMG-out
|
System 3 (4F6U-apo) DMG-out
|
System 5 (4F6UR65A_E66A) DMG-out
|
System 6 (4F7L) DMG-out
|
System 8 (4F7S) DMG-in
|
ΔEVDW
|
-163.0 ± 0.1 |
-160.7 ± 0.1 |
-160.0 ± 0.2 |
-148.7 ± 0.1 |
-156.3 ± 0.1 |
-176.5 ± 0.1 |
ΔEeel
|
-508.4 ± 1.0 |
-436.5 ± 1.0 |
-490.0 ± 1.5 |
-573.9 ± 1 |
-500.6 ± 0.9 |
-588.5 ± 0.9 |
ΔEGB
|
554.3 ± 0.9 |
491.1 ± 0.9 |
543.2 ± 1.4 |
613.9 ± 0.9 |
541.0 ± 0.8 |
613.9 ± 0.8 |
ΔEnp
|
-23.9 ± 0.0 |
-23.1 ± 0.0 |
-23.2 ± 0.3 |
-22.7 ± 0.0 |
-22.9 ± 0.0 |
-25.4 ± 0.0 |
ΔGtotal (Without entropy) |
-141.0± 0.2 |
-129.2 ± 0.2 |
-130.0 ± 0.3 |
-131.5 ± 0.2 |
-138.8 ± 0.2 |
-124.6 ± 0.2 |
Table 3.
Description of the studied systems.
Table 3.
Description of the studied systems.
System id. |
PDB id. |
Ligand name |
DMG conformation |
Manipulation |
1a |
4F6U |
0SR |
DMG-out |
(-) |
1b (replica) |
4F6U |
0SR |
DMG-out |
(-) |
2a |
4F6U |
0SR |
DMG-out |
Removal of CycC |
2b (replica) |
4F6U |
0SR |
DMG-out |
Removal of CycC |
3 |
4F6U |
(-) |
DMG-out |
Removal of ligand |
4 |
4F6U |
(-) |
DMG-out |
Removal of ligand and CycC |
5 |
4F6U |
0SR |
DMG-out |
CDK8 mutations: E66A, R65A |
6 |
4F7L |
0SO |
DMG-out |
(-) |
7 |
4F7L |
0SO |
DMG-out |
Removal of CycC |
8 |
4F7S |
0SW |
DMG-in |
(-) |
9 |
4F7S |
0SW |
DMG-in |
Removal of CycC |