1. Introduction
Plasmodium spp. are successful pathogens of humans and other vertebrates. These obligate intracellular parasites have complex life cycles and cause malaria through invasion and replication within host erythrocytes. Most species export a range of proteins to remodel their host cells to allow cytoadherence, immune evasion, and nutrient uptake [
1,
2]. These exported proteins are often encoded by multigene families that facilitate antigenic variation and evasion of host immunity [
3,
4]. Notably, members of these families are typically in subtelomeric regions, where active recombination produces new variants that increase diversity [
5,
6]. Most of these multigene families encode proteins that function in cytoadherence or immune evasion [
7]. Interestingly, most are also restricted to one or a small number of species. For example, the extensively studied
var genes in the
P. falciparum parasite are restricted to this human pathogen and a small number of species infecting African primates;
rif, vir,
yir, and
sicavar gene families are also each present in a limited number of
Plasmodium spp.
An important exception with a distinct function is the
clag multigene family (
cytoadherence
linked
asexual
gene), which is conserved in all examined members of the
Plasmodium genus [
8]. While the 3D7 reference line of
P. falciparum has 5 copies, other species have between 2 and over 35 copies of
clag genes [
9,
10]. Most
P. falciparum clones carry two copies on chromosome 3 (termed
clag3.1 and
clag3.2), but some lines have undergone copy number reduction to yield a single hybrid
clag3h [
11]. Interestingly, in vitro selection has also yielded a line with 3
clag3 genes [
12]. Three other copies in the 3D7 line (
clag2, clag8, and
clag9) are at subtelomeric sites on chromosomes 2, 8, and 9 respectively. Some lines carry additional
clag gene copies [
9], indicating ongoing evolution of this conserved gene family. Adding to these complexities,
clag genes undergo complicated epigenetic regulation with monoallelic expression and switching of
clag3 paralogs and variable expression of
clag genes on other chromosomes in
P. falciparum [
13].
The encoded CLAG proteins were originally proposed to function in cytoadherence based on molecular and biochemical studies of a chromosome 9 deletion event associated with loss of infected cell binding to the CD36 receptor [
14]. While some subsequent studies supported a role for CLAG9 in cytoadherence [
15], this observation was cast into doubt [
16]. Transcription in schizonts and packaging into rhoptries also has led to suggested roles in erythrocyte invasion and or formation of the parasitophorous vacuole that surrounds the intracellular parasite [
8,
17], but direct experimental evidence supporting these roles is missing.
In surprising contrast to these reports, more recent studies have instead strongly implicated a CLAG3 role in formation of the plasmodial surface anion channel (PSAC), a nutrient uptake channel at the host membrane [
18]. A role in PSAC-mediated nutrient uptake was first identified by unbiased genetic mapping studies with ISPA-28, a small molecule inhibitor that selectively blocks channels associated with the
clag3.1 gene product from the Dd2 parasite line but is inactive against channels associated with the Dd2
clag3.2 or either
clag3 paralog in other parasites [
19]. Additional genetic mapping studies using either the channel’s protease susceptibility or growth inhibition in PGIM, a nutrient-restricted medium, also mapped the two
clag3 genes [
12,
20]. Characterization of transport mutants generated by in vitro selection has revealed mutations in
clag3 or epigenetic silencing of
clag3 [
21,
22,
23], providing independent evidence for a role in nutrient uptake.
By comparison, much less is known about the other clag genes in P. falciparum. Indeed, the failure of genetic mapping and transport mutants to implicate these other paralogs could suggest that they serve unrelated roles in blood stage parasites. Here, we used molecular and biochemical studies to examine trafficking, localization and possible roles of the proteins encoded by clag2, clag8, and clag9. Our studies reveal that each CLAG traffics to rhoptries and is transferred to the next erythrocyte at invasion. Each reaches the host membrane with peripheral and integral membrane pools, as previously determined for CLAG3. Our protease susceptibility studies reveal that CLAG2, but not CLAG8 or CLAG9, is susceptible to extracellular protease, consistent with a larger variant domain amongst P. falciparum lines. Most importantly, our molecular and biochemical studies provide experimental evidence supporting contributions of each member to PSAC formation. These findings provide a novel example of gene family expansion to enable nutrient acquisition by intracellular parasites; they also provide insights into PSAC pharmacology that should guide antimalarial drug development against this essential channel.
4. Discussion
Erythrocytes infected with malaria parasites have increased permeability to diverse solutes, including anions, organic cations, and nutrients such as sugars, amino acids, and purines [
39,
40,
41,
42]. These increases were originally identified in ex vivo experiments from malarious monkeys [
43] and were primarily studied with macroscopic osmotic fragility and tracer flux methods until the turn of the century. Various mechanisms including one or more transporters, membrane leaks, a debated parasitophorous duct, and endocytosis were proposed based on these studies [
44,
45,
46]. Patch-clamp of
P. falciparum-infected erythrocytes resolved these mechanistic uncertainties by identifying the plasmodial surface anion channel (PSAC)[
18]. Subsequent patch-clamp studies suggested a number of distinct channels, which were proposed to be host-derived channels upregulated by the intracellular parasite [
47,
48,
49]. In vitro selection of transport mutants then revealed altered PSAC gating and reduced uptake of organic solutes, suggesting a role for parasite genetic elements [
50,
51]. These controversies were finally resolved through agnostic genetic mapping studies using ISPA-28, a strain-specific PSAC inhibitor identified in high-throughput screens [
19]. When combined with DNA transfection, these genetic mapping studies implicated CLAG3. Protease susceptibility studies revealed that CLAG3 is integral at the host membrane, suggesting a direct contribution to the nutrient uptake channel. Years before identification of its role in transport, biochemical studies had established that CLAG3 associated with RhopH2 and RhopH3, two conserved and unrelated parasite proteins [
52]. Subsequent molecular and cellular studies have revealed that these proteins also contribute to PSAC-mediated nutrient and ion transport [
33,
36], but the precise roles of each subunit have remained unclear.
While the link between PSAC activity and CLAG3 has been intensively studied [
19,
53], possible contributions from three paralogs encoded by
clag genes on
P. falciparum chromosomes 2, 8, and 9 (termed CLAG2, CLAG8, and CLAG9, respectively) have not been examined to date. Here, we address this deficit. Epitope tagging of each paralog revealed that each paralog is packaged in merozoite rhoptries, transferred to the next erythrocyte and trafficked to the host membrane, as previously established for CLAG3. Also like CLAG3, each paralog exists in at least two pools within infected erythrocytes: an alkaline-extractable form weakly associated with membranes and a form that is integral to membranes. Each member has a variant region some 30-40 kDa from its C-terminus, with the larger variant regions of CLAG3 and CLAG2 exposed to plasma upon insertion in the host erythrocyte membrane; CLAG8 and CLAG9 exhibit much less variation at this site and do not exhibit protease susceptibility, suggesting minimal or no exposure to plasma. Conserved hydrophobicity flanking this variant region suggests that CLAG3 and its paralogs have similar transmembrane topologies at the host membrane.
We used CRISPR/Cas9 transfection to disrupt each paralog and found that individual clag2 or clag8 knockouts exhibited modest increases in CLAG3 abundance. RT-PCR extended this finding by revealing statistically significant upregulation of clag3h, clag9, rhoph2, and rhoph3 in our CLAG2 and CLAG8 knockout lines. Clag2 transcription was also significantly increased in the CLAG8 knockout; clag8 increased in CLAG2 knockout but did not reach significance. These transcriptional changes establish that knockout of each paralog leads to compensatory changes in other genes linked to PSAC formation, supporting overlapping roles for these proteins.
These molecular changes were associated with a significantly increased permeability to PhTMA
+, an organic cation whose accumulation occurs almost exclusively via PSAC [
25,
41]. This increase might reflect an increase in PSAC copy number that results from the increase in CLAG3 abundance. Alternatively, it could reflect changes in PSAC selectivity for permeant solutes due to loss of individual CLAG paralogs that function as subunits of the channel [
24]. In either case, these findings represent important experimental evidence supporting roles of CLAG2 and CLAG8 in PSAC formation. We propose expansion of the
clag gene family to allow redundancy in formation of the essential nutrient uptake channel; this model suggests partially overlapping contributions through formation of a channel complex consisting of CLAG, RhopH2, and RhopH3 subunits.
In addition to expansion of the
clag gene family in
Plasmodium spp., diversity and redundancy are also promoted by transcriptional control through monoallelic expression and switching between the two
clag3 paralogs in most lines and variable expression of other
clags in
P. falciparum [
13,
54]. The benefits to the parasite are not well-understood but may include fine-tuning of channel-mediated nutrient uptake, as evidenced by switching from preferential expression of
clag3.2 in human infections to expression of
clag3.1 upon adaptation to in vitro culture [
23,
55]. Switching may also promote immune evasion by silencing of CLAG paralogs when an antibody response to an exposed epitope is generated [
56]. Epigenetic regulation has also been shown to permit escape from toxicity due to toxin uptake as silencing of
clag3 and/or
clag2 is the primary mechanism of acquired resistance to blasticidin S, which enters infected cells primarily via PSAC [
21,
23].
Systematic knockout of individual CLAG paralogs, as we performed, revealed several important differences. Most importantly, while each paralog is individually dispensable under standard in vitro culture conditions using nutrient-rich RPMI 1640 medium, loss of CLAG3 in the
C3hKO parasite aborts expansion in PGIM, a modified medium with more physiological levels of isoleucine, glutamine and hypoxanthine [
12]; isoleucine appears to be especially critical as it cannot be obtained through hemoglobin digestion [
57,
58]. In marked contrast,
C2KO,
C8KO and
C9KO all exhibited unabated growth in PGIM (
Figure 3A). One explanation for this preserved growth is that the higher levels of CLAG3 in most parasite clones may be quantitatively sufficient to permit sustained channel formation and nutrient uptake (
Figure 3). Another possibility is that channels formed with distinct paralogs permit preferential transport of certain nutrients and that the nutrients with altered levels in PGIM are primarily transported via CLAG3-associated channels. This second possibility parallels a proposed difference in nutrient acquisition by CLAG3.1 and CLAG3.2, the two isoforms present in most parasite clones [
55].
Another notable difference between the knockout lines is that while CLAG3 and CLAG2 both carry extracellular loops susceptible to protease treatment (
Figure 2), pronase treatment significantly compromises channel-mediated uptake in wild-type and
C2KO lines but has no clear effect on uptake by
C3hKO-infected cells (
Figure 4). This finding is consistent with a proposal that the channel pore is sterically occluded by the cut ends of the CLAG3 HVR produced by pronase treatment [
20]. In this proposed model, loss of CLAG3 in
C3hKO abolishes this steric hindrance and renders uptake protease-insensitive. If this model is eventually proven correct, our data would suggest that the shorter cut ends generated by cleavage of the smaller CLAG2 HVR may not be long enough to occlude the channel pore.
Our studies also reveal that while
C3hKO exhibits marked changes in PSAC pharmacology, disruption of each other paralog has negligible effects on block by ISG21, phloridzin, and furosemide (
Figure 5). ISG21 is particularly important because this compound is the parent compound for a recently reported hit-to-lead drug discovery project targeting PSAC [
59]. This finding may also reflect higher expression of CLAG3 in wild-type clones and preferential identification of inhibitors that block sites on associated channels. In this scenario, inhibitor screens using the
C3hKO line may reveal inhibitors that specifically interact with channels formed using CLAG2, CLAG8 and/or CLAG9. Such inhibitors could convincingly implicate these paralogs in direct formation of PSAC. They would also provide important structure-function insights into the channel pore and may be starting points for therapies targeting parasite nutrient acquisition.
Figure 1.
Paralogs exhibit stage-specific localization and trafficking similar to CLAG3. (A) Schematic showing the engineered C3-TetR line. This parasite carries a single chimeric clag3h product with a 1xHA epitope tag and conditional knockdown through TetR-DOZI interaction with a 10x aptamer sequence in the clag3h 3’UTR. (B) Indirect immunofluorescence antibody (IFA) images for double-tagged parasites. While anti-HA recognizes CLAG3, anti-FLAG recognizes a separate CLAG protein in each row, as indicated with a schematic to the left. Note that each paralog co-localizes with CLAG3 in rhoptries at the schizont stage (puncta, left panels) and that each is exported to the host membrane at the trophozoite stage (right). The parental control is not recognized by either antibody (bottom row). Scale bars, 5 µm.
Figure 1.
Paralogs exhibit stage-specific localization and trafficking similar to CLAG3. (A) Schematic showing the engineered C3-TetR line. This parasite carries a single chimeric clag3h product with a 1xHA epitope tag and conditional knockdown through TetR-DOZI interaction with a 10x aptamer sequence in the clag3h 3’UTR. (B) Indirect immunofluorescence antibody (IFA) images for double-tagged parasites. While anti-HA recognizes CLAG3, anti-FLAG recognizes a separate CLAG protein in each row, as indicated with a schematic to the left. Note that each paralog co-localizes with CLAG3 in rhoptries at the schizont stage (puncta, left panels) and that each is exported to the host membrane at the trophozoite stage (right). The parental control is not recognized by either antibody (bottom row). Scale bars, 5 µm.
Figure 2.
Two-state behavior and similar topologies at the host membrane. (A) Immunoblots using anti-CLAG3 antibody (first panel) or anti-FLAG antibody (all other panels) showing that indicated CLAG paralogs are minimally released upon hypotonic lysis (sol). Alkaline treatment reveals both extractable and integral membrane fractions (CO3= and membr, respectively). Samples with and without pronase E treatment of intact cells are shown to determine each paralog’s susceptibility to extracellular protease treatment. (B) Alignment of the variant regions from top to bottom: CLAG3.1 and CLAG3.2, CLAG2, CLAG8, and CLAG9. Each group contains divergent P. falciparum lines and the C3-TetR line used in these studies. Ruler above each group reflects residue number in the reference 3D7 clone. In each group, highly variant residues are highlighted in grey, while identical and conserved residues are in red and blue, respectively. Note, that CLAG3 has the largest HVR and that other paralogs have distinct degrees of variation at this site. (C) Posterior probability plots for transmembrane (TM) domain prediction for each paralog (solid black line) over a region that includes the HVR (red dash). Note the high probability for a TM distal to each HVR, consistent with membrane partitioning of the protease-induced cleavage product in CLAG3 and CLAG2. Each protein also likely has one or more transmembrane domains proximal to the HVR. Ruler at bottom indicates residue position from the unprocessed N-terminus of each paralog. (D) Schematic showing model of similar topologies at the host membrane. Variant, surface exposed sites are shown in red. Notice the smaller, less exposed surface loop on CLAG9.
Figure 2.
Two-state behavior and similar topologies at the host membrane. (A) Immunoblots using anti-CLAG3 antibody (first panel) or anti-FLAG antibody (all other panels) showing that indicated CLAG paralogs are minimally released upon hypotonic lysis (sol). Alkaline treatment reveals both extractable and integral membrane fractions (CO3= and membr, respectively). Samples with and without pronase E treatment of intact cells are shown to determine each paralog’s susceptibility to extracellular protease treatment. (B) Alignment of the variant regions from top to bottom: CLAG3.1 and CLAG3.2, CLAG2, CLAG8, and CLAG9. Each group contains divergent P. falciparum lines and the C3-TetR line used in these studies. Ruler above each group reflects residue number in the reference 3D7 clone. In each group, highly variant residues are highlighted in grey, while identical and conserved residues are in red and blue, respectively. Note, that CLAG3 has the largest HVR and that other paralogs have distinct degrees of variation at this site. (C) Posterior probability plots for transmembrane (TM) domain prediction for each paralog (solid black line) over a region that includes the HVR (red dash). Note the high probability for a TM distal to each HVR, consistent with membrane partitioning of the protease-induced cleavage product in CLAG3 and CLAG2. Each protein also likely has one or more transmembrane domains proximal to the HVR. Ruler at bottom indicates residue position from the unprocessed N-terminus of each paralog. (D) Schematic showing model of similar topologies at the host membrane. Variant, surface exposed sites are shown in red. Notice the smaller, less exposed surface loop on CLAG9.
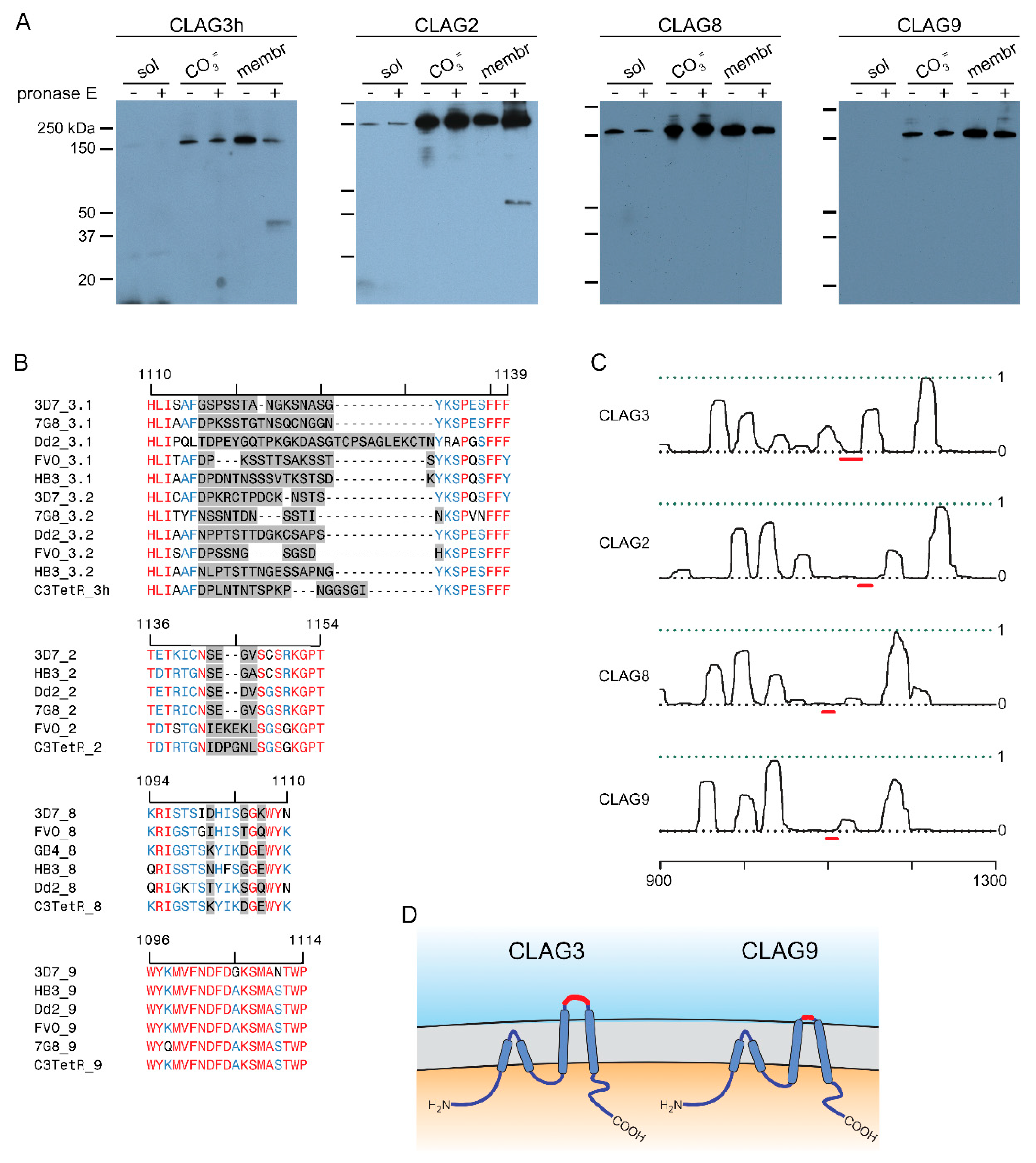
Figure 3.
Growth rates and compensatory changes in each CLAG knockout line. (A) Mean ± S.E.M. expansion of wild-type and CLAG knockout cultures over 5 days in indicated media. Black bars, wild-type KC5; red, C3hKO; blue, C2KO; dark yellow, C8KO; grey, C9KO. n = 3 independent trials each. (B) Immunoblots using total trophozoite-infected cell lysates from indicated parasites probed with anti-CLAG3 (top) and anti-aldolase as a loading control (bottom). (C) Mean ± S.E.M. ImageJ quantification of band intensities from immunoblots as in panel B after correction for the aldolase loading control band intensity and normalization of WT to 1.0. n = 3 trials each. (D) Mean ± S.E.M. normalized expression of indicated clag and rhoph genes in the WT (black) and CLAG knockout lines (blue, C2KO; dark yellow, C8KO; grey, C9KO), calculated according to 2-ΔCt using PF07_0073 as an internal loading control. n = 4 trials each. For all panels, asterisks indicate P < 0.05, one-way ANOVA with Dunnett’s multiple comparisons test.
Figure 3.
Growth rates and compensatory changes in each CLAG knockout line. (A) Mean ± S.E.M. expansion of wild-type and CLAG knockout cultures over 5 days in indicated media. Black bars, wild-type KC5; red, C3hKO; blue, C2KO; dark yellow, C8KO; grey, C9KO. n = 3 independent trials each. (B) Immunoblots using total trophozoite-infected cell lysates from indicated parasites probed with anti-CLAG3 (top) and anti-aldolase as a loading control (bottom). (C) Mean ± S.E.M. ImageJ quantification of band intensities from immunoblots as in panel B after correction for the aldolase loading control band intensity and normalization of WT to 1.0. n = 3 trials each. (D) Mean ± S.E.M. normalized expression of indicated clag and rhoph genes in the WT (black) and CLAG knockout lines (blue, C2KO; dark yellow, C8KO; grey, C9KO), calculated according to 2-ΔCt using PF07_0073 as an internal loading control. n = 4 trials each. For all panels, asterisks indicate P < 0.05, one-way ANOVA with Dunnett’s multiple comparisons test.
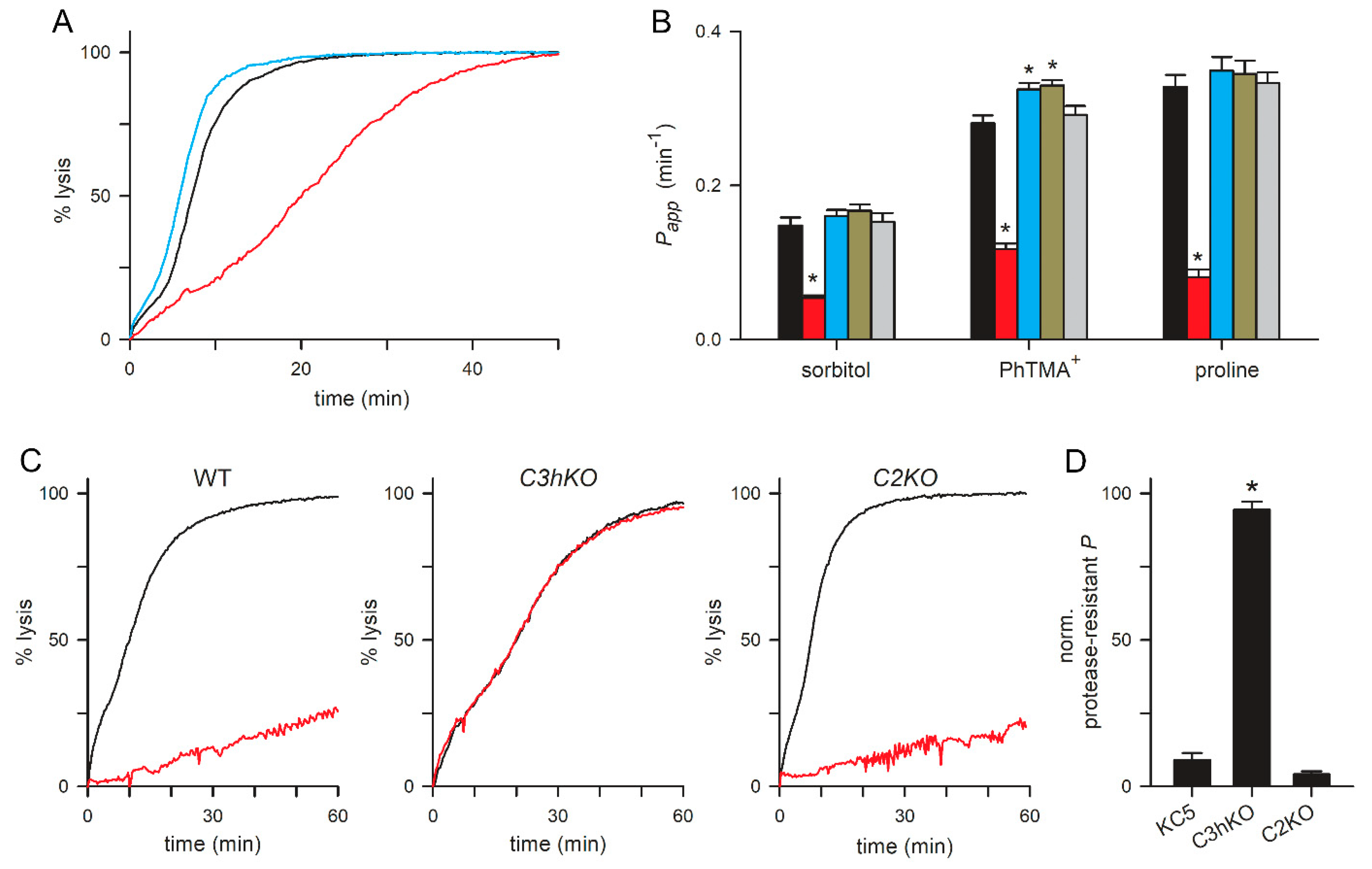
Figure 4.
PSAC-mediated transport in each CLAG knockout line. (A) Sorbitol-induced osmotic lysis kinetics for wildtype, C3hKO, and C2KO (black, red and blue traces, respectively). Note, the slower kinetic and increased time to 50% lysis for C3hKO but not for C2KO relative to the wildtype parent. (B) Mean ± S.E.M. permeabilities of indicated solutes, calculated as the reciprocal of the time to 50% lysis from osmotic lysis kinetics experiments. Black bars, wild-type KC5; red, C3hKO; blue, C2KO; dark yellow, C8KO; grey, C9KO. n = 3-8 independent trials for sorbitol and PhTMA+; 2-5 trials for proline. Asterisks, P < 0.05. (C) Sorbitol-induced lysis kinetics for indicated clones without and with pronase E pretreatment (black and red traces, respectively). Note that C3hKO’s reduced sorbitol permeability is not susceptible to proteolytic treatment, in contrast to the wildtype and C2KO. (D) Mean ± S.E.M. reduction in permeability upon pronase E treatment, normalized to 100% for no effect. Asterisk, P < 0.0001.
Figure 4.
PSAC-mediated transport in each CLAG knockout line. (A) Sorbitol-induced osmotic lysis kinetics for wildtype, C3hKO, and C2KO (black, red and blue traces, respectively). Note, the slower kinetic and increased time to 50% lysis for C3hKO but not for C2KO relative to the wildtype parent. (B) Mean ± S.E.M. permeabilities of indicated solutes, calculated as the reciprocal of the time to 50% lysis from osmotic lysis kinetics experiments. Black bars, wild-type KC5; red, C3hKO; blue, C2KO; dark yellow, C8KO; grey, C9KO. n = 3-8 independent trials for sorbitol and PhTMA+; 2-5 trials for proline. Asterisks, P < 0.05. (C) Sorbitol-induced lysis kinetics for indicated clones without and with pronase E pretreatment (black and red traces, respectively). Note that C3hKO’s reduced sorbitol permeability is not susceptible to proteolytic treatment, in contrast to the wildtype and C2KO. (D) Mean ± S.E.M. reduction in permeability upon pronase E treatment, normalized to 100% for no effect. Asterisk, P < 0.0001.
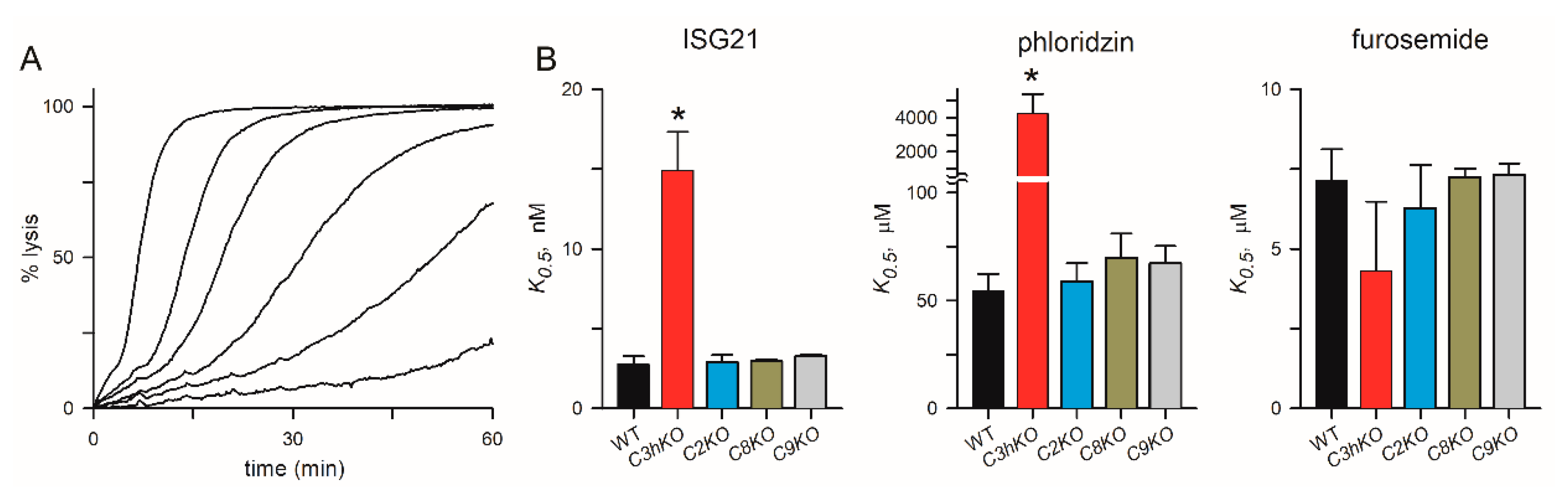
Figure 5.
Channel pharmacology in CLAG knockout lines. (A) Sorbitol-induced lysis kinetics for the KC5 wild-type line with 0, 2.5, 5, 10, 20, and 50 nM ISG21 (left to right traces). This potent inhibitor slows sorbitol uptake in a dose-dependent manner. (B) Inhibitor K0.5 values for indicated parasite clone for ISG21, phloridzin, and furosemide (left to right panels). Asterisks indicate P < 0.0001, one-way ANOVA with Dunnett’s multiple comparisons test.
Figure 5.
Channel pharmacology in CLAG knockout lines. (A) Sorbitol-induced lysis kinetics for the KC5 wild-type line with 0, 2.5, 5, 10, 20, and 50 nM ISG21 (left to right traces). This potent inhibitor slows sorbitol uptake in a dose-dependent manner. (B) Inhibitor K0.5 values for indicated parasite clone for ISG21, phloridzin, and furosemide (left to right panels). Asterisks indicate P < 0.0001, one-way ANOVA with Dunnett’s multiple comparisons test.