1. Introduction
Carbon nanotubes with outstanding physical properties have different promising applications in drug delivery, biosensors, renewable energy storage, solar cells, electronic materials, etc. [
1,
2]. Due to the high chemical and physical stability of CNTs, recently, they can extensively be used in the design of heterogeneous catalysts [
3,
4,
5]. Despite their unique properties, CNTs are chemically ineffective, hydrophobic, and have little activity on their own. However, after functionalization or modification of CNT with different ligands and functional groups, they become suitable substrates for the stabilization of active metal species and the preparation of heterogeneous catalysts. Various functional groups and ligands have been employed for the modification of the surface of carbon nanotubes, being ionic liquids of great importance due to their special properties such as high stability, assisting to dissolve reactants, increasing selectivity, and stabilizing metal nanoparticles. Furthermore, with the functionalization of CNT incorporating different ionic liquid moieties, the desired hydrophilicity and hydrophobicity can be modulated simply by exchanging the corresponding anion of IL [
6,
7]. However, ionic liquids are expensive and their use in large amounts is not economically worthwhile. One way to solve the problem is to support them onto solid surfaces allowing their easy recycling, and multiple uses [
8,
9].
Nitroaromatic compounds have extensive applications in different industries, explosives, pharmaceuticals, and in the synthesis of complex organic molecules. Nitroarenes such as nitrophenols are considered potent toxic, carcinogenic, and not biodegradable compounds, and their release into the environment and water is a hazard to living organisms and human being health [
10,
11]. On the other hand, reduction of nitro compounds to less toxic and useful amines is an efficient and promising method for transforming them. Compared to traditional methods, which are run in harsh acidic conditions, transition metal catalyzes the reduction of nitroarenes to corresponding amines in are very efficient, fast, and selective manner [
12,
13].
In addition, sulfur-containing organic compounds such as dibenzothiophene, present in fuel oils, are source of air pollution (acid rain generation) and engine corrosion [
14,
15]. Very recently, the governments around the world have planned to reduce the sulfur content of fuel to a very low level. Different strategies such as oxidative desulfurization [
16], biodesulfurization [
17], extractive desulfurization [
18] and catalytic hydrodesulfurization (HDS) [
19,
20] have been performed for lowering sulfur concentration in fuel oils. Among them, catalytic HDS processes in the presence of heterogeneous catalyst and hydrogen sources is the most commonly method for producing cleaner fuels. However, this method operates using harsh reaction condition such as high temperature and high pressure with parallel deactivation of the catalyst which has encouraged the researchers to design of new catalysts for this purpose.
In this work, we are preparing a new nitrogen-enriched carbon nanotube for the immobilization of Pd species for the application in two very important reactions namely reduction nitroarenes and desulfurization of dibenzothiophene.
2. Results and Discussions
For the preparation of the catalyst, initially, imidazole was allowed to react with propargyl bromide, and resulting 1-(prop-2-yn-1-yl)-1
H-imidazole (
A) was reacted with 1,2-dibromopropane to produce IL(
B). The multiwall carbon nanotube was treated with
m-CPBA to produce epoxy-functionalized CNT (
1). Next, CNT-epoxy was reacted with sodium azide followed by a reaction azido-CNT (
2) with IL(
B) under CuAAC to produce (
3). Furthermore, IL-modified CNT was reacted with sodium azide and resulting compound (
4) was treated with 1-(prop-2-yn-1-yl)-1
H-imidazole under CuAAC (click reaction) to produce the nitrogen-enriched CNT (
5). Finally, modified CNT was mixed with Na
2PdCl
4 to afford a CNT-supported palladium catalyst (6) which was referred as CNT-TZ-IL@Pd throughout the text (
Scheme 1).
The physical morphology and structure of the prepared materials were characterized by different techniques. Transmission electron microscopy (TEM) images of CNT-TZ-IL@Pd showed the presence of uniform carbon nanotubes and also the presence of some Pd NPs which could be generated in the presence of nitrogenous CNT (
Figure 1). Furthermore, the typical entangled morphology was clearly observed in the scanning electron microscopy (SEM) images of CNT@IL-Pd (
Figure 2).
An energy-dispersive X-ray analysis (EDX) also confirmed the presence of elements such as C, N, and Pd in the structure (
Figure 3).
To ensure the placement of organic compounds on the substrate, the Fourier Transform Infrared Spectroscopy (FTIR) was taken from all steps of the catalyst synthesis (
Figure 4). The results showed that in the spectrum of CNT-epoxy composition, the peak observed in the region of 1128-1139 cm
−1 is related to C-O-C stretching vibration [
21]. Also, the appearance of a sharp peak at 2120 cm
−1 in the spectra of CNT-N
3 (
2) and CNT-IL-N
3 (
4) compounds corresponded to the stretching vibration of the azide functional group, which indicates the successful azidation of the mentioned materials [
22]. Also, the peak located at 2120 cm
−1 in the spectrum of CNT-IL (
3) and CNT-TZ-IL (
5) compounds disappeared confirming the successful CuAAC-mediated click reaction between azide and alkyne. The related peaks to C=N appeared in the region of 1690-1640 cm
−1 and peaks due to the C=C of imidazole ring appeared around 1650 cm
−1, which overlaps with the surface –OH bending vibration. The observed peak at 1120 cm
−1 in both CNT-IL (
3) and CNT-TZ-IL (
5) compounds is related to the N-N stretching vibration in the triazole ring. Also, the peaks around 12940 cm
−1 were related to the symmetric and asymmetric stretching vibration of CH
2, and peaks located around 3600-3300 corresponded to the possible surface O-H stretching vibration [
22,
23,
24,
25,
26].
X-ray photoelectron spectroscopy (XPS) of CNT@Pd in C1s, N 1s and Pd 3d regions were studied (
Figure 5). XPS in C1s showed peaks located at 284.6, 285.6, 286.4, and 288.1 eV related to C-H/C=C, C-C, C-N, C-O-C, C=O/-C=N
+ forms of carbon [
27]. Peaks centered at 399.2, 400.6, and 401.8 eV confirm N=N, C-N, and
+NR
3 forms of nitrogen [
28]. Also, XPS of the Pd 3d region showed a doublet at 339.2 (Pd 3d3/2) and 344.5 eV (Pd 3d5/2) due to the presence of Pd(II) species [
29].
Thermal gravimetric analysis (TGA) of CNT, modified CNT (
3), and (
5) in temperatures between 25-700 °C were carefully surveyed (
Figure 6). TGA obtained from CNT-IL (
3) showed two major weight losses in the temperature range of 150-350 and 350-420 °C. The first weight reduction (21%) was attributed to the destruction of organic groups placed on the carbon nanotube surface. The second weight loss of 57% was attributed to the destruction of the CNT structure. Analogously for sample (
5), a 16% and a 57% were detected for the same ranges of the temperature, respectively. According to this data, and considering quantitative yield in all the steps required for the preparation of carbon materials (
3) and (
5), the functionalization was estimated in 0.94 and 0.70%, respectively.
X-ray diffraction analysis (XRD) of CNT-TZ-IL@Pd showed Bragg reflection related to carbon nanotubes in the region in 2θ = 25, 43, and 53 [
30]. Considering that Pd is present in the ionic form, the corresponding peaks related to Pd did not appear (
Figure 7).
Very uniform dispersion of Pd on CNT-IL was confirmed by EDX and elemental mapping images (
Figure 8).
Next, the application of the prepared catalyst was assessed in the reduction of nitroarenes. For this purpose, the reduction of 4-nitrotoluene was selected as a model reaction, and the effect of different factors on the reaction was studied. Results showed that using 0.01 mol% catalyst, NaBH
4 as the reducing agent, in solvents such as EtOH, H
2O, and H
2O:EtOH mixture, quantitative yields were achieved (
Table 1, entries 1-3). However, other solvents as ethyl acetate, acetonitrile, DMSO, DMF or THF, gave poor or low yields (
Table 1, entries 4-8), therefore, H
2O was selected as the most appropriate solvent. Other reducing agents such as formic acid, ammonium formate, glycerol, isopropyl alcohol, and hydrazine in H
2O gave poor yields (
Table 1, entries 9-13). Using a 0.005 mol% of the catalyst, the chemical yield was not altered. However, an important lowering of the yield (61%) was obtained employing a 0.003 mol% of catalyst loading (
Table 1, entries 14 and 15). Consequently, the optimum reaction conditions were H
2O as a solvent, NaBH
4 as a reducing agent, and 0.005 mol% catalyst as the suitable catalyst amount.
Next, the catalytic activity of CNT-TZ-IL@Pd (
6) was studied in the reduction of structurally different nitroarenes to corresponding amines. Results showed that reductions of aromatic nitro compounds having both electron-donating and electron-withdrawing groups proceeded very well in short reaction times. In the case of nitroarenes having a formyl group both carbonyl and nitro functional groups were reduced to alcohol and amine, respectively, while carbonyl groups of ketones, esters, and amide were intact (
Table 2, entries 25-30). The result of the high chemoselectivity leaving the ketone unaltered is a very important feature of this transformation. Reductions of heterocyclic nitroarenes were performed very well and the corresponding amines were obtained in excellent yields (
Table 2, entries 30 and 31). Pharmaceutically active nitroarenes having higher molecular weights were reacted under longer reaction times, in the presence of TPGS-750-M, as a surfactant and THF as a co-solvent (
Table 2, entries 32-35).
Finally, the environmental factor (E) for the reduction of the 4-nitrobromobenzene compound was obtained according to Shelden’s method [
31]. The value of the E factor for this reaction was determined about 4.1, which according to Sheldon’s classification range of E factors, this catalytic system is suitable for industrial use.
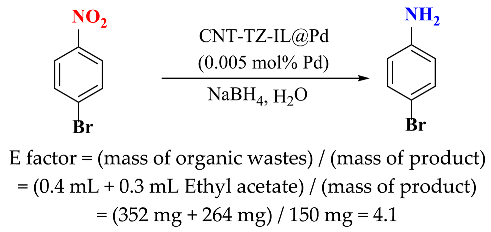
Next catalytic activity of CNT-TZ-IL was studied in the catalytic hydro-desulfurization reaction of dibenzothiophene (DBT). For this purpose, the effect of different hydrogen sources, reaction time, and temperature were studied. Results showed desulfurization of DBT (0.2 mmol) in the presence of 0.01 mol% catalyst and DBT 10 mmol gave poor yields and a 17% conversion was achieved. Increasing the amount of NaBH
4 to 20 mmol, the yield was improved to 65% (
Figure 9a). Also, results showed that increasing reaction temperature to 100 and 130 °C did not improve the reaction yield, while decreasing the temperature reaction to room temperature the yield decreased to 5% (
Figure 9b).
Next, we studied the effect of other reducing agents such as formic acid, isopropanol, and tetralin in the hydrodesulfurization of DBT. Results indicated that formic acid and isopropanol afforded very poor conversion while 80% conversion was obtained using tetralin as a hydrogen source (
Figure 10a). Finally, yields were improved to 93% conversion at 130 °C.
One of the most important aspects of heterogeneous catalysts is the possibility of recovering the catalyst from the reaction media and reusing it in successive cycles. In this regard, the recyclability of the CNT-TZ-IL@Pd catalyst for the reduction reaction of 4-nitrotoluene under optimal reaction conditions was investigated. After the completion of the reaction, the catalyst was separated from the reaction mixture using a centrifuge, washed with water and ethyl acetate, and used again in the same reaction with the addition of the raw materials. The results showed that this catalyst was recycled and reused up to 20 steps without a significant decrease in catalytic activity (
Figure 11). The recyclability of the catalyst was also studied in the hydro-desulfurization of DBT using tetralin as the hydrogen source and results indicated that the catalyst can be recyclable for 4 times without decreasing of efficiency.
The structure of the reused catalyst after 20 runs was studied with different analyses such as TEM, XPS, EDX, and XRD.
It was interesting that the TEM images of the recycled catalyst after 20th run showed the presence of palladium nanoparticles with an average size of 1.6 nm, which were uniformly dispersed on the surface of the modified CNT (
Figure 12). The presence of palladium nanoparticles was generated, possibly, by the use of sodium borohydride as reductant in the reaction process of reduction of nitro compounds. In addition, the presence of abundant nitrogen atoms in the substrate stabilized produced palladium nanoparticles.
The presence of Pd nanoparticles was further confirmed by XRD by appearing peaks at 2Ɵ = 40.1, 46.8, 68.2, and 82.2 [
30] (
Figure 13).
Figure 11.
XRD of reused catalyst in the reduction of 4-nitrotoluene.
Figure 11.
XRD of reused catalyst in the reduction of 4-nitrotoluene.
EDX analysis was studied on the recycled CNT-TZ-IL@Pd catalyst, the results showed that the structure of the catalyst was preserved and the patterns were very similar to the original catalyst (
Figure 14).
XPS spectra of the reused catalyst in C1s and N1s regions showed very similar patterns to the fresh catalyst, however, the XPS spectrum of Pd confirms the formation of Pd(0) appearing a new doublet peak at 335.2 and 340.6 eV (
Figure 15).