1. Introduction
Traumatic brain injury (TBI) affects approximately 60 million people globally [
1] and 2.8 million people in the United States each year [
2], with a rate of 759 TBIs for every 100,000 individuals reported globally in 2016[
3]. The annual number of TBIs is likely even higher as less severe TBIs often go unreported. This life-altering event can produce impairments in motor, cognitive, sensory, and affective function that can persist chronically[
4] precipitating significant costs to patients, families, and communities. Moreover, secondary elevations in intracranial pressure (ICP) to levels at or above 20mmHg following TBI can increase TBI-induced morbidity and mortality[
5,
6]. The current treatments and strategies for managing ICP elevation following a TBI are limited, and the most aggressive treatment involves invasive craniectomies [
5]. While we have some indications of the pathological changes induced by elevated ICP following TBI, these changes, particularly the diffuse pathologies that are associated with TBI, are difficult to investigate in human patients and the molecular cascades involved remain nebulous. We, therefore, utilize the central fluid percussion injury (CFPI) model in rodents, which replicates the diffuse pathologies induced by TBI, paired with specific manipulations of ICP [
7].
Previous studies from our lab using this CFPI model in adult male rats indicated that elevated ICP to 20mmHg was associated with long-term effects, including neuronal cell loss and increased sensory sensitivity at 4w following CFPI and ICP elevation, that were not seen following injury alone[
7]. Additionally, acute neuronal membrane disruption was nearly doubled with secondary intracranial pressure elevation hours following TBI[
7,
8]. Neuronal membrane disruption refers to the perturbation of the neuronal plasma membrane and is visualized using cell impermeable tracers, including dextran conjugated with fluorescent labels. Membrane disruption has been demonstrated to occur acutely and sub-acutely in vitro and in vivo in mice, rats, and swine following TBI and spinal cord injury[
7,
8,
9,
10,
11,
12,
13,
14,
15,
16,
17,
18,
19]. Recently, it was found that neuronal membrane disruption in the lateral neocortex occurs biphasicaly, in which there is significant membrane disruption sub-acutely (6hr-3d post CFPI) that is reduced at 1w post-CFPI and reemerges at more chronic timepoints (2w-4w post CFPI) [
20]
.
Previous investigations indicated that Cathepsin B (Cath B), a lysosomal cysteine protease, may play a role in the pathological progression of neuronal membrane disruption. It was shown that Cath B localizes out of the lysosomal compartment and into the cytosol, specifically in the membrane disrupted neurons at 2w and 4w post-injury [
8,
21]. Cath B has been known to participate in various cell damage and death processes [
22,
23,
24,
25,
26,
27,
28,
29,
30,
31,
32,
33]. Moreover, Cath B has been implicated in the pathologies seen in other models of TBI, such as protein upregulation, lysosomal permeability, cell death, and behavioral deficits [
34,
35,
36].
Thus, the focus of this study was to evaluate the effects of Cath B inhibition on neuronal membrane disruption 2w following injury and ICP elevation. This was accomplished using adult male rats continuously treated with the Cath B inhibitor, CA-074 methyl ester (CA-074Me), or the vehicle for CA-074Me, 10% dimethylsulfoxide (DMSO) in sterile saline, for 2w following either 1) sham injury, 2) a CFPI, or 3) a CFPI with subsequent ICP elevation to 20mmHg. We found that Cath B does not appear to be a direct driver of membrane disruption at 2w following CFPI; however, Cath B may play a role in TBI-induced and ICP exacerbated sensory hypersensitivity, warranting further investigations. We further found that 10% DMSO reliably reduced neuronal membrane disruption.
2. Materials and Methods
2.1. Animals
Experiments were conducted using protocols in accordance with the Virginia Commonwealth University institutional ethical guidelines concerning the care and use of laboratory animals (Institutional Animal Care and Use Committee, Virginia Commonwealth University), which adhere to regulations including, but not limited to, those set forth in the Guide for the Care and Use of Laboratory Animals, 8th Edition (National Research Council). Animals were housed in individual cages on a 12h light-dark cycle with free access to food and water. A total of 78 adult male Sprague-Dawley rats weighing 320–420g, n=36 for protein analyses and n=36 for histological assessments were used for this study. There were 12 animals in each group (1) Sham injury with 10% DMSO infusion, (2) Sham injury with CA-074Me infusion, (3) TBI with 10% DMSO infusion, (4) TBI with CA-074 infusion, (5) TBI+ ICP elevation with 10% DMSO infusion, and (6) TBI+ ICP elevation with CA-074Me infusion (n = 6/group for histological assessments and n=6/group for molecular assessments). Our a priori exclusion criteria included weight loss of more than 20% or gross brain pathology (contusion, subdural hematoma, or gross tissue loss). Six animals met exclusion criteria in this study and were excluded. All surgeries were conducted by the same surgeon during the same times of day to reduce variability. Animal injury state and drug infusions were randomly determined using a random number generator and prepared by a separate investigator from the surgeon.
2.2. Surgical Preparation and Injury Induction
Animals were anesthetized with 4% isoflurane in 30% O
2 and 70% room air and ventilated with 2% isoflurane in 30% O
2 and 70% room air throughout the duration of the surgery, injury, and post-injury physiological monitoring. Heart rate, respiratory rate, and blood oxygenation were monitored via a hind-paw pulse oximetry sensor (STARR Life Sciences, Oakmont, PA) for the duration of anesthesia, except during the induction of injury. Body temperature was maintained at 37°C with a rectal thermometer connected to a feedback-controlled heating pad (Harvard Apparatus, Holliston, MA). All animals were placed in a stereotaxic frame (David Kopf Instruments, Tujunga, CA). A midline incision was made, followed by a 4.8mm circular craniectomy made along the sagittal suture midway between bregma and lambda for placement of the injury hub. A 2mm burr hole was also drilled in the left parietal bone overlying the left lateral ventricle (0.8mm posterior, 1.3mm lateral, and 2.5-3mm ventral to bregma) through which a 25-gauge needle, connected to a pressure transducer and micro-infusion pump (11 Elite syringe pump; Harvard Apparatus) via sterile saline-filled PE50 tubing, was placed into the left lateral ventricle. Appropriate placement was verified via a 1.3μl/min infusion of sterile saline within the closed fluid-pressure system during needle placement with the drop in pressure indicating breach of the lateral ventricle [
8,
10]. This method of validating ventricle cannulation does not increase the intracranial pressure (ICP). The needle was held in the ventricle for at least 5min while recording pre-injury ICP. After this pre-injury reading, the needle was slowly removed, and the burr hole was covered with bone wax before preparation for sham or CFPI [
7,
37]. Briefly, a Leur-Loc syringe hub was affixed to the craniectomy site, and dental acrylic (methyl-methacrylate; Hygenic Corp., Akron, OH) was applied around the hub and allowed to harden. Anesthetized animals were removed from the stereotaxic frame and injured with a fluid pulse of 2.05±0.16 atmospheres and ~22msec. The pressure pulse was measured by a transducer affixed to the injury device and displayed on an oscilloscope (Tektronix, Beaverton, OR). Immediately after the injury, the animal was reconnected to the ventilator and physiologic monitoring device and the hub and dental acrylic were removed en bloc. Surgifoam was placed over the craniectomy/injury. The animal was then replaced in the stereotaxic device and the ICP probe was reinserted into the lateral ventricle, as described above, for post-injury ICP monitoring. At 15 minutes following injury, animals were given 0.9mg/kg buprenorphine-HCl slow release (Bup-HCl-SR) subcutaneous (SQ) as an analgesic and then monitored until 1 hour following injury. Monitoring of ICP was done for both sham and TBI only animals. For animals in the TBI and 20mmHg ICP elevation group, at 15min post-CFPI ICP was elevated to 20mmHg via infusion of sterile normal saline at 1.3-13µl/minute using the micro-infusion Pump 11 Elite syringe pump controlled by the experimenter. Once 20mmHg was achieved, ICP was maintained at 20mmHg until 1 hour after injury. At 1hr post-CFPI and ICP monitoring or elevation, animals underwent drug or vehicle bolus infusion, followed by subcutaneous implantation of a mini-osmotic pump (Alzet; Model 2002). The animals were then sutured, treated with lidocaine and triple-antibiotic ointment, recovered, and returned to a clean home cage. Identical surgical procedures were followed for sham-injured animals, without release of the pendulum to induce injury [
7,
20].
2.3. Drug Preparation and Infusion Administration
The solid form of Cath B inhibitor, CA-074 methyl ester (CA-074Me; MedChemExpress, Cat#: HY-100350, Monmouth Junction, NJ), was stored at -20°C until reconstituted in 100% dimethylsulfoxide (DMSO) at a concentration of 100 µg/µL. The 31µL reconstituted aliquots were stored at -80°C. Prior to surgery, an investigator separate from the surgeon used a random number generator to determine the animal’s injury and inhibitor infusion group. For CA-074Me infusion animals, an aliquot was defrosted and diluted in sterile 0.9% saline to a final concentration of 10µg/µl by an investigator separate from the surgeon. The solution (CA-074Me in 10% DMSO-sterile saline or 10% DMSO-sterile saline) was pipetted into tubes labeled with the date of the procedure to maintain surgeon blinding and stored at 37°C. Sterile preparation of the 250 µL mini-osmotic pump (Alzet; Model 2002) with catheter tube and brain cannula was performed by the surgeon the day prior to surgery following infusion blinding. The prepared pump was placed in a sterile tube with saline to initiate osmotic pressure and then incubated overnight at 37°C alongside the tube containing the bolus infusion. One hour following injury, the 12.5µL bolus was infused into the left lateral ventricle. Following bolus infusion, the caudal end of the midline incision was blunt dissected to lift the skin over the shoulder blades allowing for the implantation of the osmotic pump and brain infusion cannula. The cannula was placed into the burr hole for lateral ventricle using a stereotaxic cannula holder (0.8mm posterior, 1.3mm lateral, and 2.5-3mm ventral to bregma). The cannula was held in place with cyanoacrylate. The animals were then sutured, treated with lidocaine and triple-antibiotic ointment, recovered, and returned to a clean home cage. The pump infused at a rate of 0.5µL/hr for the entire 2w post-injury period.
2.4. Tracer Infusion
One hour prior to sacrifice at 2w post-sham or CFPI, tagged dextran (0.6mg/25 µg in sterile 0.9% saline; ~1.6mg/kg) was infused into the lateral ventricle in the animals being prepared for histological assessment (n=6/group) as described previously[
8,
20]. Briefly, the bur hole overlying the left lateral ventricle was reopened and another bur hole was drilled over the right lateral ventricle (0.8mm posterior, 1.3mm lateral, and 2.5-3mm ventral to bregma). 12.5µl of 10kDa dextran conjugated to 488-Alexa Fluor (Cat#: D22910, Invitrogen, Carlsbad, CA) was infused into the left then right lateral ventricle at 1.3µl/min with continuous ICP monitoring. The tracer was allowed to diffuse throughout the parenchyma for 1hr prior to transcardial perfusion.
2.5. Tissue Processing
At 2w post-injury, the animals were injected with 150mg/kg euthanasia-III solution (Henry Schein, Dublin, OH), then underwent transcardial perfusion with cold 0.9% saline. In half the animals(n=6/group), both left and right lateral neocortices were dissected and frozen for protein expression and activity assessments (n=36). Animals used for histological assessments (n=6/group; n=36) underwent the aforementioned euthanasia process, transcardial perfusion of cold 0.9% saline followed by a switch in transcardial perfusate to 4% paraformaldehyde/ 0.2% glutaraldehyde in Millonig’s buffer (136mM sodium phosphate monobasic/109mM sodium hydroxide) to fix the brain for subsequent immunohistochemical processing and analysis. After transcardial perfusion, the brains were removed, post-fixed for 24-48hr, then sectioned coronally at a thickness of 40µm in 0.1M phosphate buffer with a vibratome (Leica, Banockburn, IL) from the level of bregma to ~4.0mm posterior to bregma. Sections were collected serially in 12 well-plates and stored in Millonig’s buffer at 4°C. A random starting well (wells 1–12) was selected using a random number generator and four serial sections were used for histological analyses. All histological analyses were restricted to layers V and VI of the lateral somatosensory neocortex extending from the area lateral to CA1 to the area lateral to CA3 of the hippocampus.
2.6. Quantification of Cathepsin B Activity
Portions of the dissected left and right lateral neocortices and livers of the sham, TBI, and TBI+ICP animals were mechanically homogenized in 50μM citric acid at a pH 6.0, spun at 12,000xg at 4°C for 10 minutes, and the supernatant of the whole homogenate was collected. Protein concentrations were measured using a NanoDrop Lite (Thermo Fisher Scientific, Wilmington, DE) and Cath B activity was measured in a 96-well plate, each well containing 2x Assay Buffer (100mM sodium acetate pH 5.5, 2mM EDTA, 200mM sodium chloride, 8mM DTT), 2μg of neocortex whole homogenate, and 1mM Z-Phe-Arg-7-amino-4-(trifluoromethyl) coumarin (ZFR-AMC), a substrate for cysteine proteases that fluoresces upon cleavage by Cath B [
21,
34,
35,
38]. The plate was read on a PHERAstar microplate reader (BMG Labtech, Cary, NC, USA) at 60-minutes post-substrate addition at 365/450 nm excitation/emission. Each sample was loaded in triplicate per plate and was run on three independent plates to reduce pipetting and run-to-run variability biasing the results. A positive control well with 5ng of purified human liver Cath B and a negative control well with only assay buffer and substrate were included on every plate. The raw arbitrary fluorescent values depicting activity were measured and expressed as fold increase compared to the negative control, which was designated as 0-fold activity.
2.7. Western Blotting
Left lateral neocortices of (1) Sham injury with 10% DMSO infusion, (2) Sham injury with CA-074Me infusion, (3) TBI with 10% DMSO infusion, (4) TBI with CA-074Me infusion, (5) TBI+ ICP elevation with 10% DMSO infusion, and (6) TBI+ ICP elevation with CA-074Me infusion (n = 6/group), were homogenized in western lysis buffer (150 mM NaCl, 50 mM Tris pH 8.0, 1% Triton) and protease inhibitor cocktail (AEBSF 10.4mM, Aprotinin 8μM, Bestatin 400μM, E-64 140μM, Leupeptin 8μM, Pepstatin A 150μM, Cat#: P8340, Sigma, Saint Louis, MO). Protein concentrations were measured using a BCA Protein Assay (Pierce Biotechnology, Thermo Fisher Scientific, Rockford, IL, Cat#: 23227) according to manufacturer’s instructions and read on a PHAREstar plate reader (BMG-Lab tech, Cary NC, USA). Protein (20μg for Cath B, 5μg for Bcl-XL, 10μg for Bak and AIF) was boiled for 10min in 50mM dithiothreitol (Cat#: 1610610; Bio-Rad; Hercules, CA), 2x Laemelli loading buffer (Cat#: 1610737; Bio-Rad; Hercules, CA) and run at 200 Volts for 30min on Mini-PROTEAN TGX Stain-Free 4-20% precast polyacrylamide gels (Cat#: 4568096; Bio-Rad, Hercules, CA). Protein was transferred onto 0.45μm PVDF membranes using a Bio-Rad Transblot Turbo transfer system set to the Mixed Molecular Weight manufacturer setting (1.3-2.5 Amps, 25 Volts for 7min). Western blotting was done on an iBind Flex apparatus (Invitrogen, Carlsbad, CA) using primary antibodies rabbit anti-Cath B (1:1000; Cat#: 31718S, RRID:AB_2687580), rabbit anti-Bak (1:1000; Cat#: 12105S, RRID:AB_2716685), rabbit anti-Bcl-xl (1:1000; Cat#: 2764S, RRID:AB_2228008) or rabbit anti-AIF (1:1000; Cat#: 5318S, RRID:AB_10634755). All primary antibodies were purchased from Cell Signaling Technology, Danvers, MA. Secondary antibody anti-rabbit-HRP (1:5000; Cat#: 111-035-003; Jackson ImmunoResearch Laboratories, West Grove, PA, RRID:AB_2313567) was used for all assays. Total protein (Stain Free) and chemiluminescent images were taken on a ChemiDoc imaging system (BioRad) holding the exposure time consistent for each protein assessed. Densitometric analyses of Cath B, AIF, Bak, and Bcl-XL were performed in ImageJ (National Institutes of Health; Bethesda, MD). Cath B, AIF, Bak and Bcl-XL protein bands were normalized to total loaded protein then to a naive control sample that was included in all runs. All western blots were run in triplicates on three separate gels to reduce run-to-run variability biasing the results.
2.8. Membrane Disruption and Total Cell Count Analysis
Consistent with previous studies, we assessed the potential for neuronal membrane disruption via the utilization of 10kDa dextran, which is impermeable to cells with intact membranes [
8]. Fluorescently tagged dextran-containing cells, indicative of membrane perturbation, could be visualized via fluorescent microscopy without further processing. To identify individual neurons, four tissue sections from (1) Sham injury with 10% DMSO treatment, (2) Sham injury with CA-074Me treatment, (3) TBI with 10% DMSO treatment, (4) TBI with CA-074 treatment, (5) TBI+ ICP elevation with 10% DMSO, and (6) TBI+ ICP elevation with CA-074Me groups (n= 6/group) were stained with 1:500 dilution of NeuroTrace 435/455 blue fluorescent Nissl Stain (Life Technologies, Eugene, OR, Cat# N21479). The tissue was mounted onto slides using Vectashield Vibrance mounting medium (Cat# H-1700; Vector Laboratories, Burlingame, CA). Sections were imaged with fluorescent optical sectioning microscopy using a Keyence BZ-X800 microscope (Keyence Corporation of America, Itasca, IL, USA). Quantitative analysis was performed as described previously [
7]. Briefly, images (20-35/animal) of the left neocortical region of interest were taken at 40X magnification in a systematically random fashion by a blinded investigator using NeuroTrace to verify focus. Image acquisition settings were held constant for comparable regions (layer V or VI) for all groups analyzed. Analyses of neurons exhibiting dextran uptake were performed using the ImageJ cell counting plug-in and verified cellular identity using the NeuroTrace staining. Dextran containing neurons were quantified for each image and averaged for each animal.
To assess for cell loss the total number of NeuroTrace stained neurons were counted using the Hybrid Cell Counter function of the Keyence BZ-X800 Analyzer software. Briefly, images were set to simple thresholding with uniform brightness and no smoothing. Auto thresholding was used to highlight the NeuroTrace positive label followed by watershed cell separation and removal of cell bodies smaller than 15μm in diameter to exclude smaller glial cells and fragments of cell bodies from the analysis.
2.9. Cellular Cathepsin B Localization Analysis
Fluorescently tagged dextran-containing cells, indicative of membrane disruption, could be visualized via fluorescent microscopy without further processing. To visualize Cath B, three sections/ animal and 6 animals/ group were labeled with Cath B. Tissue sections were blocked with 5% normal goat serum (NGS), 2% bovine serum albumin (BSA), and permeabilized with 1.5% triton-X for 2h. This was followed by immunolabeling using primary antibodies rabbit anti-Cath B (1:700; Cat#: 31718; Cell Signaling Technology; Danvers, MA; RRID:AB_2687580) and mouse anti-NeuN (1:500; Cat#: MAB377; MilliporeSigma; Temecula, CA, RRID:AB_2298772). Tissue was incubated in secondary antibodies Alexa-647 conjugated goat anti-mouse(1:700; Cat#: A32728; Life Technologies, Rockford, IL; RRID:AB_10563566) and Alexa-568 conjugated goat anti-rabbit (1:800; Cat#: A11036; Life Technologies, Eugene, OR; RRID:AB_2534102), and the tissue was mounted onto slides using Vectashield hardset mounting medium with 4’,6-diamidino-2-phenylindole (DAPI) (Cat#: H-1500; Vector Laboratories, Burlingame, CA). Quantitative analysis was performed as described previously[
8,
20]. Briefly, 5-6 images from the 3 sections/animal of the left neocortical region of interest were taken at 40X magnification using a Keyence BZ-X800 microscope (Keyence Corporation of America, Itasca, IL, USA) in a systematically random fashion by an investigator blinded to the animal group using the dextran tag to verify images included neurons with and without membrane disruption. Image acquisition settings were held constant for comparable regions (layer V or VI) and 488-dextran for all groups analyzed. The images including 488-dextran, DAPI, and Cath B were analyzed in FIJI (Image J). DAPI was used to identify nuclei of cells containing and not containing 488 dextran. Ten non-disrupted and ten membrane disrupted cells assessed in each image (n=5-6 images/ animal). If a total of 10 neurons that were membrane disrupted could not be found an equal number of membrane disrupted and non-disrupted neurons were captured in that image; n=6 animals/group (1) Sham injury with 10% DMSO treatment, n=373 cells, (2) Sham injury with CA-074Me treatment, n=424 cells, (3) TBI with 10% DMSO treatment, n=442 cells,(4) TBI with CA-074Me treatment, n=480 cells, (5) TBI+ ICP elevation with 10% DMSO, n=497 cells, and (6) TBI+ ICP elevation with CA-074Me, n=432 cells. Cells were classified by Cath B appearance of punctate (intra-lysosomal) or diffuse (extra-lysosomal) as has been done previously [
8,
21]. The localization of Cathepsin B was expressed as the percentage of the total membrane disrupted or non-disruption neuronal population assessed in each experimental group that demonstrated punctate (lysosomal) Cath B localization.
2.10. Whisker Nuisance Task
Hypersensitivity in the whisker barrels was assessed using the whisker nuisance task (WNT)[
7,
39,
40]. Briefly, animals were assessed prior to injury and at 2w post sham or CFPI by an investigator blind to animal group. For this assessment the animal was placed inside a clean plastic open field lined with a chux pad and allowed to acclimate for 5 minutes. A wooden applicator stick was brushed along the rat’s whiskers on each side, for a 5-minute trial period followed by 1min rest between each of 3 total trials. During these trials the following behaviors were scored on a scale of 0 to 2 (0 = normal, 1 = exhibits some nuisance behavior, 2 = exhibits profound nuisance behavior): (1) movement, (2) stance and body position, (3) breathing, (4) whisker position, (5) whisker response, (6) evading stimulation, (7) response to stick presentation, (8) grooming, (9) ear position, (10) sniffing, (11) fur ruffling, and (12) urination/defecation (Supplemental
Table S1). The individual behavior scores for each animal were summed for each trial with the highest possible score being 24, and the sums were averaged for the three trials. A higher WNT score indicates more pronounced agitation/sensitivity.
2.11. Statistics
Data were tested for normality prior to utilizing parametric or non-parametric assessments, which were conducted using SPSS (IBM Corporation, Armonk, NY) software. Animal numbers for each group were determined by an a priori power analysis using effect size and variability previously observed in the lab when assessing pathology between sham and injured groups using the CFPI model, an alpha =0.05, and a power of 80%. Two-way or one-way analysis of variance (ANOVA) and Bonferroni post hoc tests were performed for all between-group analyses. Statistical significance was set to p<0.05. Data are reported as mean ± standard error of the mean (SEM).
4. Discussion
Overall, the findings from this study show that CA-074Me, when given as a bolus followed by continuous osmotic pump infusion into the left ventricle, successfully inhibits Cath B activity in the left and right lateral neocortex (
Figure 1). Cath B activity was not significantly different between injury categories. The protein quantifications for Bak, Bcl-XL, and AIF also revealed no changes regarding CA-074Me treatment (
Figure 3,
Figure 4 and
Figure 5). However, there was a small but significant increase in the protein levels of the lower band of Cath B in the groups given CA-074Me. (
Figure 2). This could indicate a compensatory mechanism in which cells express increased levels of Cath B in the face of reduced Cath B activity, however, further investigations regarding expression and degradation of this lower Cath B band would be needed to rigorously investigate this possibility. Our histological assessments uncovered no cell loss in the lateral neocortex in layers V and VI in any group (
Figure 7). We also did not observe a difference in the percentage of neuronal membrane disruption in any group regardless of injury or inhibitor infusion (
Figure 8). Assessments of Cath B localization recapitulated the reduced punctate Cath B localization in membrane disrupted neurons compared to non-disrupted neurons (
Figure 10), as has been shown previously [
21]. Inhibition of Cath B also significantly altered Cath B localization (
Figure 10). Somatosensory hypersensitivity, which was increased with injury and injury with elevated ICP did appear to be reduced by CA-074Me infusion in animals sustaining CFPI with secondary ICP elevation (
Figure 11).
The neuronal membrane disruption findings in the current study, particularly in the 10% DMSO vehicle control groups were unanticipated based on previous studies. We previously found that while sham injury precipitated very little neuronal membrane disruption, approximately 20% of neurons were membrane disrupted at 6hr following CFPI in adult male Fisher rats [
8]. The percentage of neurons sustaining membrane disruption doubled to 40% in rats with naturally high ICP above 20mmHg following CFPI [
8]. A follow-up study using Sprague-Dawley rats found similar percentages of neuronal membrane disruption in which injured rats had ~25% membrane disrupted neurons and injury with ICP elevation to 20mmHg demonstrated ~62% membrane disrupted neurons[
7]. A study investigating the time course of membrane disruption from 6h to 4w following a CFPI in male Sprague Dawley rats also found significantly higher percentages of neuronal membrane disruption following injury both acutely and weeks following injury [
20]. Therefore, it was surprising to see no increase in neuronal membrane disruption following CFPI and no exacerbation with secondary ICP elevation (Figure. 8). However, while DMSO is mostly noted for its potentially toxic effects if used in high concentrations[
42,
43,
44], DMSO has also been shown to have a neuroprotective effects. In a study conducted by DiGiorgio and colleagues, using the lateral fluid percussion model (LFPI) in rats, they tested drugs alpha-tocopherol and curcumin, with DMSO as the vehicle control as well as including saline controls[
45]. In their assessments of cell damage/death they found a significant reduction in Fluoro-Jade staining in all drug treated groups suspended in DMSO vehicle, including the DMSO only group which was not observed in the saline LFPI controls [
45]. Earlier preclinical studies saw that intravenous administration of this amphipathic molecule precipitated a reduction in ICP [
46,
47]. DMSO was also assessed in the clinic as a potential treatment for intractable ICP elevation and was shown to rapidly reduce ICP[
42,
43,
44,
48]. However, it was challenging to administer clinically as the DMSO tended to break down IV tubing. In addition, DMSO could lead to hemolysis and hypernatremia/hyperosmolarity, which counteracted any positive impacts of lowering ICP [
42,
43,
44].
Biophysical assessments of DMSO have demonstrated increased permeability/flexibility in membrane dynamics [
49,
50]; however, the full effects of DMSO on cellular membranes is not fully understood. DMSO’s effects on membranes was initially interrogated using molecular simulations in which Notman et al., found increased membrane flexibility which was postulated to be linked to formation of water pores[
50]. Another group arrived at similar conclusions using atomic simulations [
49]. These simulations were eventually tested in DC-3F Chinese hamster lung fibroblast cells exposed to different percentages of DMSO
(Ménorval et al., 2012). Membrane undulations, but not membrane permeability, was documented with low doses of DMSO[
51]. With DMSO concentrations closer to 10-20% small membrane blebs were seen in which Ca2+ diffused into the cells, however, there was no increased diffusion of the larger (630Da) cell impermeable Oxazole Yellow (Yo-Pro-1) molecule for this concentration of DMSO [
51]. Increased Yo-Pro-1 diffusion into cells was not significantly higher until ≥25% DMSO was administered to cells[
51]. Indeed, addition of 5% DMSO to transected guinea pig spinal cords axons reduced permeability of axonal membranes to a cell impermeable tracer, even under conditions that the group had previously determined to significantly hinder membrane resealing[
52]. In the current study it may be possible that DMSO altered membrane flexibility thereby promoting membrane resealing by 2w post-injury, which could explain the lower amounts of membrane disruption we observed compared to previous studies [
7,
20,
40].
We did, however, observe whisker sensory sensitivity increases following CFPI that was exacerbated by ICP elevations to 20mmHg in our 10% DMSO infused group, consistent with previously published studies [
7,
40]. Notably, CA-074Me administration in animals sustaining TBI and secondary ICP elevation lowered sensory hypersensitivity to levels consistent with the TBI only groups infused with either 10% DMSO or CA-074Me (
Figure 11). Previous groups found similar rescues in deficits with Cath B inhibition. Mice administered CA-074 prior to a focal TBI showed recovery of motor function and spatial learning compared to vehicle treated animals[
36]. Another study in which Cath B was knocked out of mice sustaining a focal TBI, or inhibited via administration of E64d, a cysteine protease inhibitor, observed better motor behavior in animals with absent or inhibited Cath B compared to controls[
35]. Based on these studies and our current finding, it is possible that Cath B inhibition may play a role in the exacerbation of whisker hypersensitivity following secondary ICP elevation. Overall, while the mechanisms behind membrane disruption are not directly coupled with Cath B, Cath B seems to have a role in the exacerbation of behavioral morbidities following TBI and secondary ICP elevation, offering a molecular target in treating the after-effects of elevated ICP following injury. Therefore, further study into this possibility is warranted.
Figure 1.
Cathepsin B (Cath B) activity was reduced following 2w of CA-074Me infusion into the left lateral ventricle. Cath B activity was significantly decreased in the left and right lateral neocortex following CA-074Me infusion (filled boxes) compared to 10% DMSO control (vehicle; open boxes). Box and whisker graph depicting the average fluorescent intensity indicating Cath B activity in the right and left side of the lateral neocortex and the liver of sham injured control animals (n=12; grey opened boxes for n=6 saline treated animals and filled boxes for n=6 CA-074Me treated animals), animals sustaining a TBI only (n=13; light blue opened boxes for n=6 saline treated animals and filled boxes for n=7 CA-074Me treated animals), or animals sustaining a TBI followed by secondary ICP elevation (n=12; dark blue opened boxes for n=6 saline treated animals and filled boxes for n=6 CA-074Me treated animals). Note that Cath B activity in the liver was significantly higher than that in the cortex regardless of injury group. Additionally, ICP infusion of CA-074Me did not impact Cath B activity in the liver; however, CA-074Me infusion did significantly lower Cath B activity in the left and right cortex, compared to DMSO controls. * p<0.05 compared to injury type-matched vehicle control, # p<0.05 compared to injury type-matched liver. Mean ± S.E.M.
Figure 1.
Cathepsin B (Cath B) activity was reduced following 2w of CA-074Me infusion into the left lateral ventricle. Cath B activity was significantly decreased in the left and right lateral neocortex following CA-074Me infusion (filled boxes) compared to 10% DMSO control (vehicle; open boxes). Box and whisker graph depicting the average fluorescent intensity indicating Cath B activity in the right and left side of the lateral neocortex and the liver of sham injured control animals (n=12; grey opened boxes for n=6 saline treated animals and filled boxes for n=6 CA-074Me treated animals), animals sustaining a TBI only (n=13; light blue opened boxes for n=6 saline treated animals and filled boxes for n=7 CA-074Me treated animals), or animals sustaining a TBI followed by secondary ICP elevation (n=12; dark blue opened boxes for n=6 saline treated animals and filled boxes for n=6 CA-074Me treated animals). Note that Cath B activity in the liver was significantly higher than that in the cortex regardless of injury group. Additionally, ICP infusion of CA-074Me did not impact Cath B activity in the liver; however, CA-074Me infusion did significantly lower Cath B activity in the left and right cortex, compared to DMSO controls. * p<0.05 compared to injury type-matched vehicle control, # p<0.05 compared to injury type-matched liver. Mean ± S.E.M.
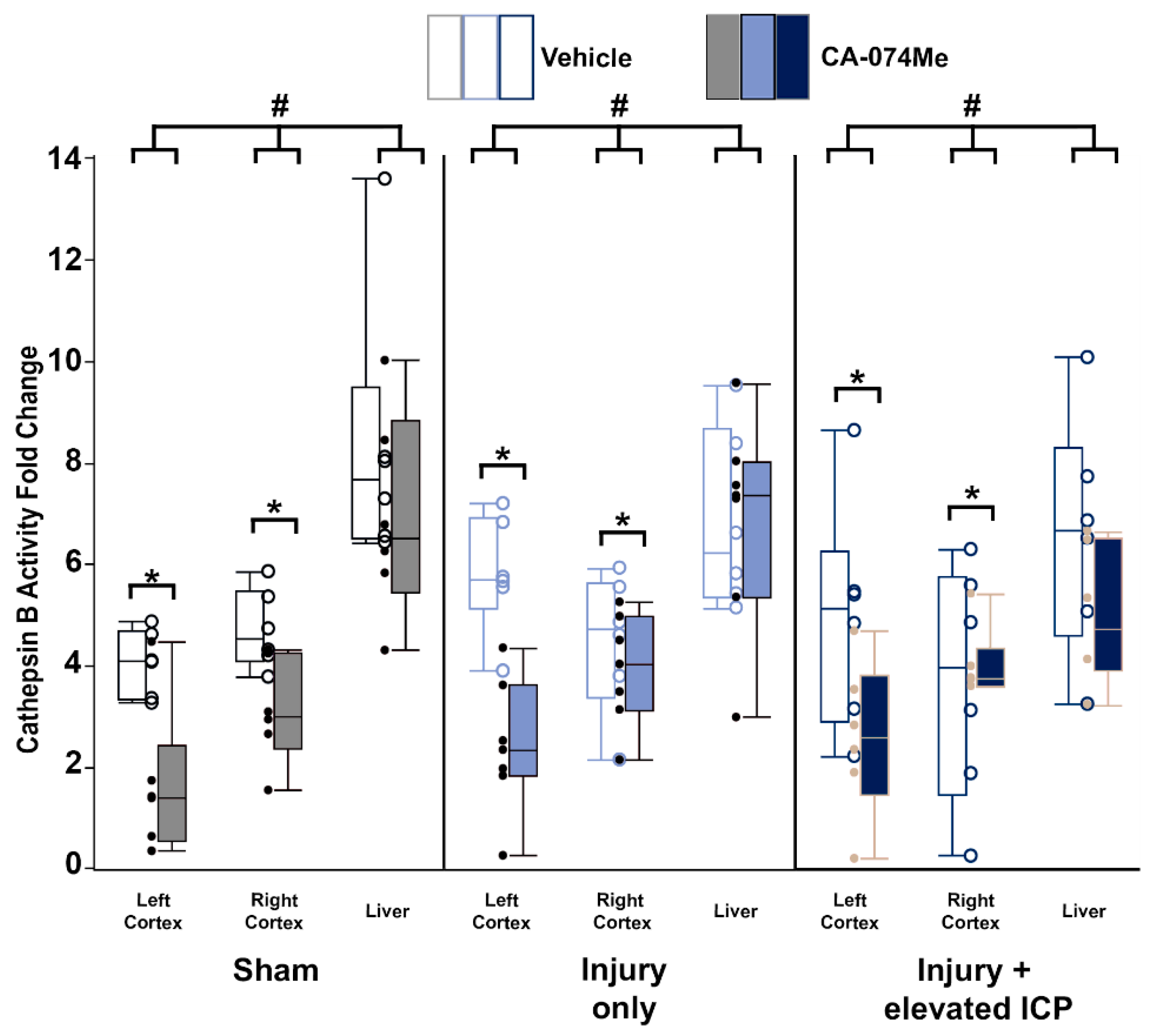
Figure 2.
Cathepsin B (Cath B) protein levels were slightly increased in animals infused with CA-074Me. (A) Representative chemiluminescent blot image of the two mature Cath B bands at 24/27 kDa, which was normalized to (B) total protein. Box and whisker graphs depicting average (n=6/group) quantities of (C) total Cath B protein, (D) the upper band of the Cath B doublet, and (E) the lower band of the Cath B doublet for sham injured animals (grey boxes), animals sustaining a CFPI (light blue boxes), and animals sustaining both CFPI and secondary ICP elevation (dark blue boxes) followed by a 2w ICF infusion of either 10% DMSO (vehicle, left half of graphs) or the Cath B inhibitor, CA-074Me, (right half of graphs). Amount of Cath B for each case was calculated as a percentage compared to a consistent naïve control that was run on all membranes. While there were no signifiant differences found for total Cath B protein or the upper band of the observed doublet, there was a significant increase in Cath B levels of the lower band of the doublet in animals infused with CA-074Me compared to their vehicle counterparts. * p<0.05 compared to vehicle. Mean ± S.E.M.
Figure 2.
Cathepsin B (Cath B) protein levels were slightly increased in animals infused with CA-074Me. (A) Representative chemiluminescent blot image of the two mature Cath B bands at 24/27 kDa, which was normalized to (B) total protein. Box and whisker graphs depicting average (n=6/group) quantities of (C) total Cath B protein, (D) the upper band of the Cath B doublet, and (E) the lower band of the Cath B doublet for sham injured animals (grey boxes), animals sustaining a CFPI (light blue boxes), and animals sustaining both CFPI and secondary ICP elevation (dark blue boxes) followed by a 2w ICF infusion of either 10% DMSO (vehicle, left half of graphs) or the Cath B inhibitor, CA-074Me, (right half of graphs). Amount of Cath B for each case was calculated as a percentage compared to a consistent naïve control that was run on all membranes. While there were no signifiant differences found for total Cath B protein or the upper band of the observed doublet, there was a significant increase in Cath B levels of the lower band of the doublet in animals infused with CA-074Me compared to their vehicle counterparts. * p<0.05 compared to vehicle. Mean ± S.E.M.
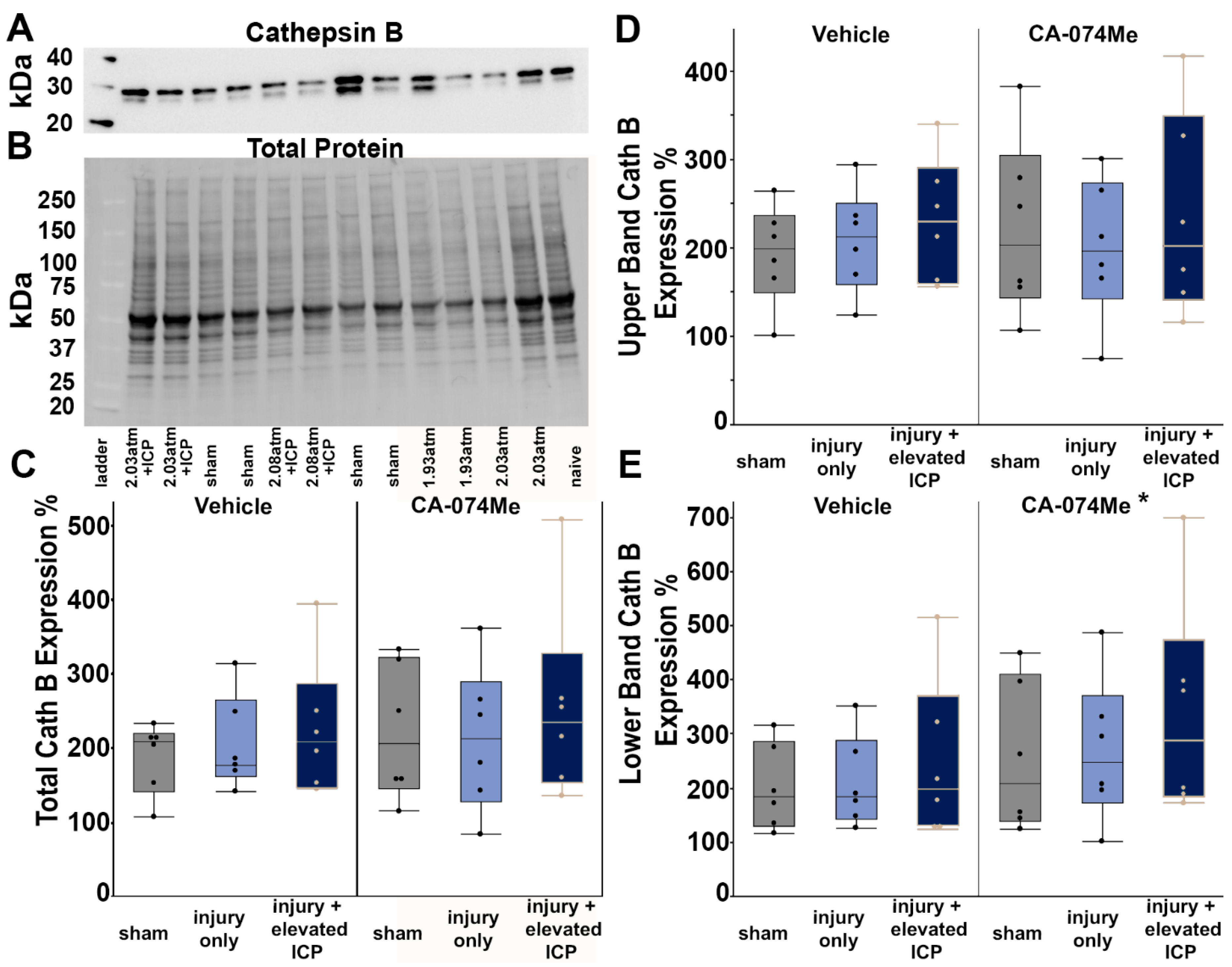
Figure 3.
Protein levels of Bcl-XL were unchanged regardless of injury or infusion group. (A) Representative chemiluminescent blot image of Bcl-XL at 30 kDa, which was normalized to (B) total protein. (C) Box and whisker graph depicting average (n=6/group) quantities of Bcl-XL protein for sham injured animals (grey boxes), animals sustaining a CFPI (light blue boxes), and animals sustaining both CFPI and secondary ICP elevation (dark blue boxes) followed by a 2w ICF infusion of either 10% DMSO (vehicle, left half of graph) or the Cath B inhibitor, CA-074Me, (right half of graph). Amount of Bcl-XL for each case was calculated as a percentage compared to a consistent naïve control that was run on all membranes. Mean ± S.E.M.
Figure 3.
Protein levels of Bcl-XL were unchanged regardless of injury or infusion group. (A) Representative chemiluminescent blot image of Bcl-XL at 30 kDa, which was normalized to (B) total protein. (C) Box and whisker graph depicting average (n=6/group) quantities of Bcl-XL protein for sham injured animals (grey boxes), animals sustaining a CFPI (light blue boxes), and animals sustaining both CFPI and secondary ICP elevation (dark blue boxes) followed by a 2w ICF infusion of either 10% DMSO (vehicle, left half of graph) or the Cath B inhibitor, CA-074Me, (right half of graph). Amount of Bcl-XL for each case was calculated as a percentage compared to a consistent naïve control that was run on all membranes. Mean ± S.E.M.
Figure 4.
Protein quantification of BAK revealed no differences in the protein quantity regardless of group. (A) Representative chemiluminescent blot image of BAK at 25kDa, which was normalized to (B) total protein. (C) Box and whisker graph depicting average (n=6/group) quantities of BAK protein for sham injured animals (grey boxes), animals sustaining a CFPI (light blue boxes), and animals sustaining both CFPI and secondary ICP elevation (dark blue boxes) followed by a 2w ICF infusion of either 10% DMSO (vehicle, left half of graph) or the Cath B inhibitor, CA-074Me (right half of graph). Amount of BAK for each case was calculated as a percentage compared to a consistent naïve control that was run on all membranes. Mean ± S.E.M.
Figure 4.
Protein quantification of BAK revealed no differences in the protein quantity regardless of group. (A) Representative chemiluminescent blot image of BAK at 25kDa, which was normalized to (B) total protein. (C) Box and whisker graph depicting average (n=6/group) quantities of BAK protein for sham injured animals (grey boxes), animals sustaining a CFPI (light blue boxes), and animals sustaining both CFPI and secondary ICP elevation (dark blue boxes) followed by a 2w ICF infusion of either 10% DMSO (vehicle, left half of graph) or the Cath B inhibitor, CA-074Me (right half of graph). Amount of BAK for each case was calculated as a percentage compared to a consistent naïve control that was run on all membranes. Mean ± S.E.M.
Figure 5.
Protein quantification of AIF revealed no differences in the protein quantity regardless of group. (A) Representative chemiluminescent blot image of the two observed AIF bands at ~67/72kDa, which was normalized to (B) total protein. Box and whisker graphs depicting average (n=6/group) quantities of (C) the upper band of the AIF doublet and (D) the lower band of the AIF doublet for sham injured animals (grey boxes), animals sustaining a CFPI (light blue boxes), and animals sustaining both CFPI and secondary ICP elevation (dark blue boxes) followed by a 2w ICV infusion of either 10% DMSO (vehicle, left half of graphs) or the Cath B inhibitor, CA-074Me, (right half of graphs). Amount of AIF for each case was calculated as a percentage compared to a consistent naïve control that was run on all membranes. Mean ± S.E.M.
Figure 5.
Protein quantification of AIF revealed no differences in the protein quantity regardless of group. (A) Representative chemiluminescent blot image of the two observed AIF bands at ~67/72kDa, which was normalized to (B) total protein. Box and whisker graphs depicting average (n=6/group) quantities of (C) the upper band of the AIF doublet and (D) the lower band of the AIF doublet for sham injured animals (grey boxes), animals sustaining a CFPI (light blue boxes), and animals sustaining both CFPI and secondary ICP elevation (dark blue boxes) followed by a 2w ICV infusion of either 10% DMSO (vehicle, left half of graphs) or the Cath B inhibitor, CA-074Me, (right half of graphs). Amount of AIF for each case was calculated as a percentage compared to a consistent naïve control that was run on all membranes. Mean ± S.E.M.
Figure 6.
Representative fluorescent micrographs of membrane disruption in sham injured animals (Sham, A and D), animals sustaining a CFPI (TBI, B and E), and animals sustaining a CFPI followed by secondary ICP elevation (TBI+ 20 mmHg ICP elevation, C and F) paired with 2w of ICV infusion of 10% DMSO (vehicle, A-C) or the Cath B inhibitor, CA-074Me (D-F). The left panel in blue depicts NeuroTrace Nissl stained cells and the middle panel in green depicts cells containing a cell impermeable Alexa fluor-488-tagged dextran (AF-Dextran-488). The right panel is the overlay of the NeuroTrace and membrane disrupted dextran images. The arrow heads indicate representative membrane disrupted neurons. Scale bar 20μm.
Figure 6.
Representative fluorescent micrographs of membrane disruption in sham injured animals (Sham, A and D), animals sustaining a CFPI (TBI, B and E), and animals sustaining a CFPI followed by secondary ICP elevation (TBI+ 20 mmHg ICP elevation, C and F) paired with 2w of ICV infusion of 10% DMSO (vehicle, A-C) or the Cath B inhibitor, CA-074Me (D-F). The left panel in blue depicts NeuroTrace Nissl stained cells and the middle panel in green depicts cells containing a cell impermeable Alexa fluor-488-tagged dextran (AF-Dextran-488). The right panel is the overlay of the NeuroTrace and membrane disrupted dextran images. The arrow heads indicate representative membrane disrupted neurons. Scale bar 20μm.
Figure 7.
The total number of cells in the lateral neocortex layers V and VI is unaffected across injury and infusion groups. Bar graph depicting the average number of fluorescent NeuroTrace Nissl-stained neurons in sham injured animals (grey boxes), animals sustaining a CFPI (light blue boxes), and animals sustaining both CFPI and secondary ICP elevation (dark blue boxes) followed by a 2w ICV infusion of either 10% DMSO (vehicle, left half of graphs) or the Cath B inhibitor, CA-074Me, (right half of graphs). The mean number of cells was quantified per unit area (0.098 mm2) and averaged for each animal (n=6/group). Mean ± S.E.M.
Figure 7.
The total number of cells in the lateral neocortex layers V and VI is unaffected across injury and infusion groups. Bar graph depicting the average number of fluorescent NeuroTrace Nissl-stained neurons in sham injured animals (grey boxes), animals sustaining a CFPI (light blue boxes), and animals sustaining both CFPI and secondary ICP elevation (dark blue boxes) followed by a 2w ICV infusion of either 10% DMSO (vehicle, left half of graphs) or the Cath B inhibitor, CA-074Me, (right half of graphs). The mean number of cells was quantified per unit area (0.098 mm2) and averaged for each animal (n=6/group). Mean ± S.E.M.
Figure 8.
There were no significant changes in membrane disruption regardless of injury or Cath B inhibitor infusion. Bar graph highlighting a consistently low average (n=6 group) percentage of membrane disrupted neurons in sham injured animals (grey boxes), animals sustaining a CFPI (light blue boxes), and animals sustaining both CFPI and secondary ICP elevation (dark blue boxes) followed by a 2w ICV infusion of either 10% DMSO (vehicle, left half of graphs) or the Cath B inhibitor, CA-074Me, (right half of graphs). Mean ± S.E.M.
Figure 8.
There were no significant changes in membrane disruption regardless of injury or Cath B inhibitor infusion. Bar graph highlighting a consistently low average (n=6 group) percentage of membrane disrupted neurons in sham injured animals (grey boxes), animals sustaining a CFPI (light blue boxes), and animals sustaining both CFPI and secondary ICP elevation (dark blue boxes) followed by a 2w ICV infusion of either 10% DMSO (vehicle, left half of graphs) or the Cath B inhibitor, CA-074Me, (right half of graphs). Mean ± S.E.M.
Figure 9.
Representative fluorescent micrographs of Cathepsin B (Cath B) localization in membrane disrupted and non-disrupted neurons within the lateral neocortex layers V and VI in sham injured animals (Sham, A and D), animals sustaining a CFPI (TBI, B and E), and animals sustaining a CFPI followed by secondary ICP elevation (TBI+ 20 mmHg ICP elevation, C and F) paired with 2w of ICV infusion of 10% DMSO (vehicle, A-C) or CA-074Me (Cath B inhibitor, D-F). The left most panel contains DAPI labeled nuclei (blue). Neurons were identified as non-disrupted or membrane disrupted based upon uptake of cell impermeable Alexa-fluor488 tagged 10kDa dextran (AF-Dextran-488; second panel in green). Immunolabeling for Cath B (third panel in red) allowed investigation of Cath B localization inside lysosomal puncta (punctate) or outside lysosomes. The right most panel is an overlay of the single channel images. Scale bar 20μm.
Figure 9.
Representative fluorescent micrographs of Cathepsin B (Cath B) localization in membrane disrupted and non-disrupted neurons within the lateral neocortex layers V and VI in sham injured animals (Sham, A and D), animals sustaining a CFPI (TBI, B and E), and animals sustaining a CFPI followed by secondary ICP elevation (TBI+ 20 mmHg ICP elevation, C and F) paired with 2w of ICV infusion of 10% DMSO (vehicle, A-C) or CA-074Me (Cath B inhibitor, D-F). The left most panel contains DAPI labeled nuclei (blue). Neurons were identified as non-disrupted or membrane disrupted based upon uptake of cell impermeable Alexa-fluor488 tagged 10kDa dextran (AF-Dextran-488; second panel in green). Immunolabeling for Cath B (third panel in red) allowed investigation of Cath B localization inside lysosomal puncta (punctate) or outside lysosomes. The right most panel is an overlay of the single channel images. Scale bar 20μm.
Figure 10.
Cathepsin B (Cath B) inhibition with CA-074Me impacted the distribution of Cath B within lysosomal puncta. Bar graph depicting the percent of neurons within the non-disrupted (left half of graph; Sham DMSO n=214 neurons [dark green bars], Sham CA-074Me n=248 [light green bars], TBI DMSO n=238 neurons [dark blue bars], TBI CA-074Me n=275 neurons [light blue bars], TBI+ICP DMSO n=226 neurons [dark pink bars], and TBI+ICP CA-074Me n=351 neurons [light pink bars]) and membrane disrupted (right half of graph; Sham DMSO n=159 neurons [dark green bars], Sham CA-074Me n=176 neurons [light green bars], TBI DMSO n=204 neurons [dark blue bars], TBI CA-074Me n=205 neurons [light blue bars], TBI+ICP DMSO n=153 neurons [dark pink bars], TBI+ICP CA-074Me n=199 neurons [light pink bars]) populations that exhibited punctate localization of Cath B. Note that Cath B localization within puncta was impacted by inhibitor infusion, injury group, and membrane disruption status of the neurons analyzed. * p<0.05 compared to non-disrupted counterpart, # p<0.05 compared to sham & CA-074Me, $ p <0.05 compared to vehicle-treated counterpart, ^ p<0.05 compared to TBI only infusion group counterpart. Mean ± S.E.M.
Figure 10.
Cathepsin B (Cath B) inhibition with CA-074Me impacted the distribution of Cath B within lysosomal puncta. Bar graph depicting the percent of neurons within the non-disrupted (left half of graph; Sham DMSO n=214 neurons [dark green bars], Sham CA-074Me n=248 [light green bars], TBI DMSO n=238 neurons [dark blue bars], TBI CA-074Me n=275 neurons [light blue bars], TBI+ICP DMSO n=226 neurons [dark pink bars], and TBI+ICP CA-074Me n=351 neurons [light pink bars]) and membrane disrupted (right half of graph; Sham DMSO n=159 neurons [dark green bars], Sham CA-074Me n=176 neurons [light green bars], TBI DMSO n=204 neurons [dark blue bars], TBI CA-074Me n=205 neurons [light blue bars], TBI+ICP DMSO n=153 neurons [dark pink bars], TBI+ICP CA-074Me n=199 neurons [light pink bars]) populations that exhibited punctate localization of Cath B. Note that Cath B localization within puncta was impacted by inhibitor infusion, injury group, and membrane disruption status of the neurons analyzed. * p<0.05 compared to non-disrupted counterpart, # p<0.05 compared to sham & CA-074Me, $ p <0.05 compared to vehicle-treated counterpart, ^ p<0.05 compared to TBI only infusion group counterpart. Mean ± S.E.M.
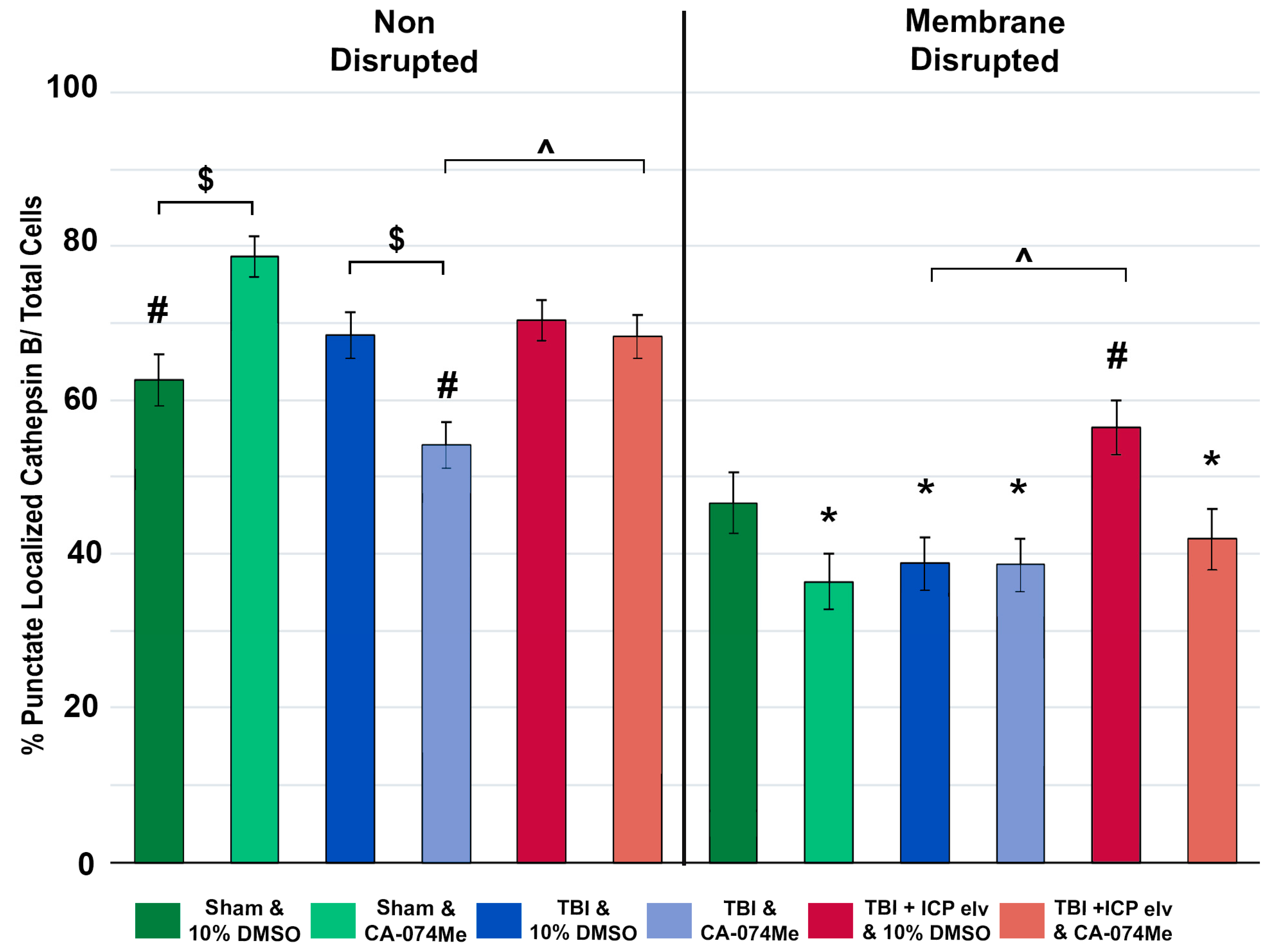
Figure 11.
Somatosensory sensitivity was impacted by injury, particularly with secondary ICP elevation, and further impacted by cathepsin B inhibition with CA-074Me. Bar graph depicting the average whisker nuisance task (WNT) score pre-injury (tan bars) and at 2w post-injury or sham (blue bars). Sham animals infused with 10% DMSO (n=11) had slightly higher WNT scores post-injury compared to sham animals infused with CA-074Me (n=13). Animals sustaining TBI infused with 10% DMSO (n=12) demonstrated similar post-injury WNT scores as TBI animals infused with CA-074Me (n=13). Injured animals with secondary ICP elevations (TBI + 20mmHg ICP) infused with CA-074ME (n=12) had lower WNT scores compared to injured and ICP elevated animals infused with 10% DMSO (n=11) resulting in an overall reduction in post-injury WNT score in animals infused with CA-074Me. * p<0.05 compared pre-injury WNT score for that group, # p<0.05 compared to sham 10% DMSO. Mean ± SEM.
Figure 11.
Somatosensory sensitivity was impacted by injury, particularly with secondary ICP elevation, and further impacted by cathepsin B inhibition with CA-074Me. Bar graph depicting the average whisker nuisance task (WNT) score pre-injury (tan bars) and at 2w post-injury or sham (blue bars). Sham animals infused with 10% DMSO (n=11) had slightly higher WNT scores post-injury compared to sham animals infused with CA-074Me (n=13). Animals sustaining TBI infused with 10% DMSO (n=12) demonstrated similar post-injury WNT scores as TBI animals infused with CA-074Me (n=13). Injured animals with secondary ICP elevations (TBI + 20mmHg ICP) infused with CA-074ME (n=12) had lower WNT scores compared to injured and ICP elevated animals infused with 10% DMSO (n=11) resulting in an overall reduction in post-injury WNT score in animals infused with CA-074Me. * p<0.05 compared pre-injury WNT score for that group, # p<0.05 compared to sham 10% DMSO. Mean ± SEM.