1. Introduction
The central nervous system (CNS) uses chemical messengers called neurotransmitters to transmit information between neurons [
1]. Many neurologic and neuropsychiatric disorders cause dysregulations of neurotransmitter function, and the neurological underpinnings of behavior can be uncovered by studying neurotransmitter dynamics [
2,
3,
4,
5,
6,
7,
8,
9]. Fast scan cyclic voltammetry (FSCV) is a powerful method to study the real-time release and reuptake of electroactive neurotransmitters. FSCV is an electrochemical method that typically uses a carbon fiber microelectrode (CFME) referenced to a Ag/AgCl electrode to detect redox currents from electroactive analytes by applying a cyclic potential waveform at high scan rates. This method utilizes the magnitude and voltage associated with the redox current peaks to estimate the concentrations and identity of an analyte, respectively [
10]. FSCV is particularly useful for the detection of monoamine neurotransmitters, such as catecholamines (dopamine (DA), norepinephrine, and epinephrine) as well as indolamines (serotonin (5-HT) and histamine) [
11,
12,
13,
14,
15,
16].
Monoamine neurotransmitters can produce reaction products following oxidation. In particular, 5-HT induces the formation of polymerizing oxidative byproducts that have a higher adsorption affinity to the CFME than 5-HT itself, disrupting electron transfer and subsequent signal detection [
15,
17,
18,
19]. This phenomenon, known as “electrochemical fouling”, is separate from “biofouling” which arises from adsorption of other biological interferents to the electrode surface such as blood, damaged tissue, etc. [
10,
20,
21,
22]. Both types of fouling can interfere with
in vivo FSCV signal detection, especially in the chronic setting where exposure to fouling agents may be prolonged. A variety of strategies have been explored to combat electrode fouling. Generally, these strategies treat both types of fouling as one phenomenon, but some groups, such as Weese et al. have shown that the two types of fouling may have unique requirements to best mitigate them. They found electrochemical fouling resistance can be achieved with electrodes that have a pristine surface, whereas biofouling resistance is achieved with functionalization with hydrophilic groups, indicating a different mechanism between the two fouling types [
17]. Generally, to resist both types of fouling, researchers have explored new carbon materials, coatings, and alternative waveform parameters. Alternative carbon materials such as carbon nanotubes (CNTs) [
17,
23,
24], nanodiamonds (NDs) [
24,
25,
26], and boron-doped diamond (BDD) [
21,
27,
28,
29,
30,
31,
32,
33,
34,
35,
36] have shown promise in reducing fouling by leveraging their unique surface characteristics, (e.g., polarity, defect sites, and carbon-bonding structures). Various coatings have been developed to reduce fouling, including Nafion, base-hydrolyzed cellulose acetate, and fibronectin [
20]. Several studies have explored adjusting waveform parameters to mitigate both biofouling and electrochemical fouling through various mechanisms [
14,
21,
37,
38,
39,
40]. Oxidative etching driven by increased switching potentials (> 1.1 V) will constantly refresh the CFME surface, which can remove fouling agents of both types, but will degrade the electrode over time [
41]. The “Jackson” N-Shaped waveform was designed to outrun and reject adsorption of the oxidative byproducts of 5-HT by altering the scan rate and potentials of the waveform to avoid the adsorption of byproducts while maintaining sensitivity and selectivity for 5-HT [
15]. Likewise, our recent report indicated that when using the Jackson waveform, the effects of biofouling were less pronounced on boron-doped diamond microelectrodes (BDDMEs) in comparison to CFMEs [
21].
Our group is developing a freestanding, all-diamond, batch fabricated BDDME (
Figure 1) as an alternative to the CFME to potentially mitigate fouling while reducing etching [
21,
28,
42]. The device is batch fabricated using a wafer-scalable process which can produce hundreds of identical microelectrodes simultaneously without the need for cumbersome manual assembly. The BDDME is a fiber-style electrode grown on silicon wafers using a combination of microwave chemical vapor deposition (CVD) to grow the BDD and hot-filament CVD to insulate the conductive core with polycrystalline diamond (PCD). This approach enables readily customizable geometries to be fabricated to meet the needs of various sensing configurations. Generally, BDD also provides several other purported benefits when used for electrochemical sensing such as a wider working potential window, stable background currents, and improved resistance to mass loss due to oxidative etching [
27,
31,
42,
43]. Many of these desirable characteristics arise from diamond’s
sp3 hybridized carbon bonding structure, which can create a tradeoff in sensitivity from the
sp2 hybridized bonding of other carbon materials [
44].
Our previous work with our BDDME includes an initial characterization [
42] and explorations into FSCV waveform development and biofouling performance [
21,
39]. Given our confirmation that the BDDME does resist biofouling relative to the CFME, we wanted to test the electrochemical fouling performance of our electrodes. Concurrently, while exploring fabrication techniques to improve the sensitivity and consistency of our devices, we chose to characterize femtosecond laser cutting as an alternative method to expose the BDD electrode site at the fiber tip. In previous work, we completed this fabrication step by physically cleaving the tip of the electrode with a scalpel. Femtosecond lasers, often used in ophthalmic surgery, offer an extremely precise, focused laser which is known for its reduced propensity to damage unintended areas surrounding the focal point through minimized thermal flux [
45]. We are also exploring the femtosecond laser for an “on-wafer” fabrication step, automating a portion of fabrication normally done by hand utilizing using motorized translation stages.
This study compared 5-HT electrochemical fouling performance between the CFME, the physically cleaved BDDME (C-BDDME), and the femtosecond laser-cut BDDME (FS-BDDME). After initially testing differences in sensitivity, the electrochemical fouling was explored for both DA and 5-HT with similar methods to previous work [
14,
17] using both a moderate concentration of 5-HT (5 µM) and a large concentration (50 µM). Additionally, we provide an analysis of these devices’ long-term stabilities to further explore the BDDME’s suitability as chronic FSCV sensor, where long term stability is especially important. We found the following: (1) The C-BDDME resists electrochemical fouling from 5-HT compared to the CFME and the FS-BDDME at both 5 and 50 µM 5-HT, (2) FS-BDDMEs have sensitivities comparable to CFMEs, but do not maintain the fouling resistance and stability of C-BDDMEs, (3) the C-BDDME has a stable background when exposed to the DA FSCV waveform at 60 Hz for 24 hours whereas the CMFE and FS-BDDME both experience changes. We intend this work to be additive to efforts toward viable, chronic neurochemical sensors.
4. Discussion
Neurochemical sensing can be a powerful way to improve the understanding and treatment of neurological behaviors and conditions. Using FSCV, real-time insight into neurochemical dynamics can be elucidated. However, there are limitations for chronic applications of this technique, including electrode fouling. Biofouling is considered as the interference of any unwanted biological material that adheres to the electrode sensing surface such as proteins, blood, soft tissue, etc. Electrochemical fouling is fouling induced by oxidative byproducts of analytes that outcompete for adsorption sites on the sensing surface. This is a well-known phenomenon affecting 5-HT detection when using the standard waveform with FSCV and CFMEs [
15,
17,
19]. In order to reduce the effects of fouling, alternative carbon materials are being explored as replacements for CFMEs. Our group has been developing an all-diamond, batch fabricated BDDME as an alternative to the traditional CFME. As an extension of our work on biofouling [
21], we tested the electrochemical fouling performance of the BDDME since it has been indicated that the two fouling types are facilitated through different mechanisms [
17]. In part, we were motivated to explore this effect by our recent findings that the BDDME exhibits a more robust and consistent response to 5-HT than DA, which is practically significant due to serotonin’s roles in neuropsychiatric disease states (
Figure 4D, S3) [
54,
55,
56,
57].
The ultimate goal of the development of our all-diamond, freestanding BDDME is to develop a chronically implantable sensor capable of neurotransmitter detection over long periods of time. However, several challenges remain, including achieving adequate sensitivity to detect physiologically relevant concentrations of neurotransmitters
in vivo, reducing fouling, and maintaining electrode stability during long-term use. The sensitivity of the BDDME is much lower than the CFME due to its ~10x smaller electroactive surface area (CFME: ~1000-1600 µm
2, BDDME: ~100-200 µm
2) as well as diamond’s less adsorptive material properties [
28,
30,
41]. Femtosecond laser cutting can be used to functionalize the BDDME to a comparable sensitivity to the CFME, which has been used for numerous
in vivo explorations [
11,
52,
57,
58,
59,
60,
61]. Laser cutting converts
sp3-bonded diamond to
sp2-bonded graphitic content, which may come at the expense of some of diamond’s desirable properties, including fouling resistance and background stability [
27,
44,
62,
63,
64]. With the FS-BDDME, this is supported by visible inspection of a dark appearance on the electrode surface in SEM images (
Figure 1F, S2), as well as its similar electrochemical characteristics to that of the CFMEs (e.g. background sizes and shapes, sensitivities to DA and 5-HT, response kinetics, and LODs). Additionally, high temperature-acid oxidation can be used to etch
sp2 bonded carbon from diamond [
68,
69]. We soaked BDDMEs in a 1:1:1 ratio of concentrated acids (
Section 2.9). A large reduction in the electrochemical backgrounds were observed with FS-BDDMEs (972.52 nA, n=2) as compared to a near zero (0.44 nA, n=3) reduction from C-BDDMEs, indicating the removal of a larger amount of
sp2-bonded carbon from the FS-BDDME (
Figure S5). Post-acid cleaned FS-BDDME backgrounds were still larger than the C-BDDME backgrounds. This may be attributed to an incomplete removal of
sp2-bonded carbon or an increase in the electroactive surface area due to the addition of microchannels from the FS-laser pulsing or a combination of both. It also appears that the graphitic content covers a larger span than just the conductive BDD, since the darkening and laser pulses (vertical channels) can be observed across the entire face of the electrodes as seen in the SEM images (
Figure 1F, S2). Given the large increase in background size and sensitivity, it is possible that laser cutting functionalizes more than just the BDD core, resulting in a substantial increase in the electroactive surface area. If so, these devices would be expected to exhibit fouling characteristics more like the CFME than the C-BDDME, aligning with the presented findings.
Electrochemical fouling is an important consideration in the development of a chronic 5-HT sensor, since the oxidative byproducts of 5-HT are known to foul the electrode surface. The FSCV potential waveform has been adapted to avoid some effects of 5-HT fouling as demonstrated by Jackson et al. (0.2 V to 1.0 V to -0.1 V to 0.2 V at 1000 Vs
-1 at 10 Hz) [
15], and expanded upon by Dunham and Venton [
14]. However, in the Dunham and Venton experiments [
14], the dopamine waveform exhibits the least fouling after 25 injections of 1 µM 5-HT using CFMEs compared to the Jackson waveform and its tested variants. In this study, we observed that the C-BDDME exhibits improved electrochemical fouling performance over the CFME, with a significantly more stable peak oxidation current response to repeated injections of 5-HT. This may be explainable by low surface adsorption due to BDD’s
sp3-bonded structure [
29,
34]. The FS-BDDME was more susceptible to 5-HT fouling than both the CFME and the C-BDDME. We observed a clear concentration-dependent fouling effect for 50 µM 5-HT compared to 5 µM 5-HT. Since 5-HT oxidation produces polymerizing radicals that form an electron-transfer-hindering film on the electrode surface [
18], increased 5-HT oxidations should be expected to cause more fouling. This effect is further supported by absence of measurable fouling to 1 µM 5-HT shown by Dunham and Venton with CFMEs [
14]. The C-BDDME maintains its resistance to fouling at higher concentrations and exhibits a slower rate of fouling than the CFME’s exponential decay. This reduced rate of decay could potentially reflect a contribution of a less absorptive surface of the C-BDDME [
21,
39,
65]. The FS-BDDME fouled less than the CFME in the 50 µM experiments and had a rate of decay more closely matched to the C-BDDME than the CFME. We speculate that with large concentrations, the FS-BDDME’s highly adsorptive graphitic content is quickly fouled while the
sp3-bonded BDD-portion retains some level of fouling resistance, leading to an “in-between” fouling performance to the CFME and C-BDDME. Optimization of the laser-cutting protocol may allow for a similar, but less pronounced “in-between” effect where the FS-BDDME maintains improvements in both sensitivity over the C-BDDME and fouling resistance compared to the CFME.
Signal stability is another important goal in chronic applications where recording is required over long periods of time. The CFME is known to be very stable over short windows (~ 90s) [
51,
52] and exhibited stable responses over a two hour window with 5-HT in our study. However, the etching behavior of the CFME [
41,
66] causes a self-cleaning, surface renewing effect and increases the number of adsorption sites on the electrode surface. In turn, this leads to increased sensitivity and a growing background current, which we observed on our 60Hz, 24-hour test. This evolution over time needs to be accounted for in the chronic setting particularly when estimating concentrations since there may be sensitivity changes [
51,
67]. Eventually, the carbon-fiber will erode away to an unusable level from consistent etching [
31,
41]. Part of the motivation to use BDD electrodes in place of the CFME is to reduce etching and maintain signal stability over longer periods of time [
31]. Our data confirmed that the C-BDDME had both a stable two-hour response repeatability, and a very minimal background shift after the 24-hour etching test. The C-BDDMEs may better maintain a stable surface without sacrificing material to etching. On the other hand, stability experiments revealed a trend toward a reduction in sensitivity over time with the FS-BDDME, likely due to etching away of the
sp2-bonded carbon created by the laser, revealing the
sp3-bonded diamond beneath. Thus, although the FS-BDDME greatly improves the sensitivity of the electrode in comparison to the C-BDDME, this benefit comes at the expense of stability. Additionally, we plan to investigate micromachining strategies and laser-cutting parameters to tune the level of
sp2 conversion of the FS-BDDME, as FS machining has been used to form nanometer-width grooves that may provide an increased electrochemically active surface area while maintaining diamond composition [
70].
As the neurochemical sensing field progresses toward improved chronic sensing capabilities, it is important to thoroughly characterize emerging technologies that intend to replace traditional devices. BDDMEs may deliver improved stability and reduced fouling, but at the expense of sensitivity. Surface-modifying techniques may recover sensitivity, but at the expense of stability. These trade-offs require further design iteration to fully leverage the benefits of the BDDME. However, it is also important to acknowledge that the BDDME presented here is not a one-to-one comparison with the CFME. The large disparity between the sensitivity of the BDDME and the CFME is affected by the electroactive surface area differences between the two devices [
24,
53]. For reference, example CVs from the CFME and the C-BDDME with currents normalized to estimated electroactive surface areas (CFME: 1250 µm
2 and C-BDDME: 150 µm
2 calculated from estimated exposed geometric surface area) are included (
Figure S6). These surface-area adjusted plots result in an approximately 10x lower response from the C-BDDME. This is contrary to the 100x smaller currents from the non-corrected data, indicating the sensitivity differences are largely due to differing electroactive surface areas. However, a better comparison would still include comparable sensing surface sizes and geometries. As next steps, we recommend increasing the electroactive surface area to increase sensitivity, while maintaining a small device size in order to minimize glial encapsulation [
71,
72,
73,
74,
75,
76]. In addition to this, refinement of the BDDME through sensitivity-increasing methods, such as exploring coatings, overoxidation, waveform development, boron-doping level, and chemometric data analysis may be viable solutions to deliver an optimized, chronic BDDME sensor with customizable, batch-fabricated architectures.
Author Contributions
Author Contributions: Conceptualization, M.L.P., B.G., J.R.S., W.L. and E.K.P.; Methodology, M.L.P., B.G., J.R.S., B.K., R.R., A.M., M.H. and E.K.P.; Formal Analysis and Data Curation, M.L.P., B.G., J.R.S., I.E.C., B.K., and E.K.P.; Resources, J.R.S., R.R., A.M., M.H., and M.F.B.; Writing—original draft preparation M.L.P., B.G., J.R.S., B.K., and E.K.P.; Writing—review and editing, M.L.P., B.G., J.R.S., B.K., A.M., M.H., R.R., M.F.B., E.K.P. and W.L.; Supervision, Project Administration, and Funding Acquisition—M.F.B., W.L. and E.K.P. All authors have read and agreed to the published version of the manuscript.
Figure 1.
BDDME fabrication and representative devices. (A.) Material and geometry pattern for silicon (Si) wafer-based chemical vapor deposition. Boron doped diamond (BDD) is grown on a Si wafer, then reactive ion etching (RIE) is used to pattern the BDD. The BDD probes are released from the Si wafer and insulating polycrystalline diamond (PCD) is grown around the BDD shanks. (B) Representative CFME tip at 900x magnification. (C.) Scanning electron microscope (SEM) image of the BDDME shank, integrated connection pad, and the sensing tip. (D) C-BDDME tip at 1500x magnification. (E.) SEM image of the sensing tip of a C-BDDME. The conductive BDD core is exposed from the PCD during fabrication by cleaving the end of the shank with a knife, or by laser-cutting the shank with a femtosecond laser. (F) FS-BDDME tip at 1500x magnification. The left column of this figure is adapted from [
21].
Figure 1.
BDDME fabrication and representative devices. (A.) Material and geometry pattern for silicon (Si) wafer-based chemical vapor deposition. Boron doped diamond (BDD) is grown on a Si wafer, then reactive ion etching (RIE) is used to pattern the BDD. The BDD probes are released from the Si wafer and insulating polycrystalline diamond (PCD) is grown around the BDD shanks. (B) Representative CFME tip at 900x magnification. (C.) Scanning electron microscope (SEM) image of the BDDME shank, integrated connection pad, and the sensing tip. (D) C-BDDME tip at 1500x magnification. (E.) SEM image of the sensing tip of a C-BDDME. The conductive BDD core is exposed from the PCD during fabrication by cleaving the end of the shank with a knife, or by laser-cutting the shank with a femtosecond laser. (F) FS-BDDME tip at 1500x magnification. The left column of this figure is adapted from [
21].
Figure 2.
Representative current versus time (I vs. t) traces and 3D color plots for the CMFE, C-BDDME, and FS-BDDME with 5-HT. (A-C) show the I vs. t plot for a representative 1st and 25th 5-HT injection of 5-HT. (D-F) depict color plots for the 1st 5-HT response, and (G-I) show color plots for the 25th.
Figure 2.
Representative current versus time (I vs. t) traces and 3D color plots for the CMFE, C-BDDME, and FS-BDDME with 5-HT. (A-C) show the I vs. t plot for a representative 1st and 25th 5-HT injection of 5-HT. (D-F) depict color plots for the 1st 5-HT response, and (G-I) show color plots for the 25th.
Figure 3.
Calibration curves. All plots include the linear regressions for the entire calibrated ranges and their corresponding line equations. Table 1 includes calculations done using the four calibrated data points with the highest linearity (CFME and FS-BDDME: 0.025-0.2 µM 5-HT, C-BDDME: 2-20 µM 5-HT) (A.) CFME calibration curve with a slope of 47.5 nAµM-1 with the concentration range of 25-1000 nM 5-HT (n = 6). (B.) C-BDDME calibration curve with a slope of 0.0914 nAµM-1 with the concentration range of 1-100 µM 5-HT (n = 4-5). (C.) FS-BDDME calibration curve with a slope of 0.0914 nAµM-1 with the concentration range of 1-100 µM 5-HT (n = 4).
Figure 3.
Calibration curves. All plots include the linear regressions for the entire calibrated ranges and their corresponding line equations. Table 1 includes calculations done using the four calibrated data points with the highest linearity (CFME and FS-BDDME: 0.025-0.2 µM 5-HT, C-BDDME: 2-20 µM 5-HT) (A.) CFME calibration curve with a slope of 47.5 nAµM-1 with the concentration range of 25-1000 nM 5-HT (n = 6). (B.) C-BDDME calibration curve with a slope of 0.0914 nAµM-1 with the concentration range of 1-100 µM 5-HT (n = 4-5). (C.) FS-BDDME calibration curve with a slope of 0.0914 nAµM-1 with the concentration range of 1-100 µM 5-HT (n = 4).
Figure 4.
Electrochemical fouling results for 5 µM 5-HT and DA. Current is represented as a percentage of the oxidation peak of the first injection. (A, D, and G) Representative (n=1) changes in cyclic voltammograms (CVs) from the 1st (black) to the 25th (pink) 5-HT bolus injection. (B, E, and H) 25 consecutive oxidation peak currents from 5 µM DA (black) and 5 µM 5-HT (pink) (n=6, 4, and 5 from top to bottom). The 5-HT fouling trajectories were significantly different between all three electrode types (P < 0.001, linear mixed model ANOVA with a Bonferroni post-hoc test). (C, F, and I) Percent changes from the 1st to the 25th injection for DA (black) and 5-HT (pink). The change in 5-HT peak current from the 1st to 25th injection was also significant for each device type using two-tailed, paired t-tests (CFME, P < 0.001), (C-BDDME, P < 0.05), and (FS-BDDME, P < 0.001). The CFME also showed a significant decrease in DA response, although it had the lowest percentage drop of 4.9 ± 1.5% (t-test, two-tailed, paired, P < 0.05).
Figure 4.
Electrochemical fouling results for 5 µM 5-HT and DA. Current is represented as a percentage of the oxidation peak of the first injection. (A, D, and G) Representative (n=1) changes in cyclic voltammograms (CVs) from the 1st (black) to the 25th (pink) 5-HT bolus injection. (B, E, and H) 25 consecutive oxidation peak currents from 5 µM DA (black) and 5 µM 5-HT (pink) (n=6, 4, and 5 from top to bottom). The 5-HT fouling trajectories were significantly different between all three electrode types (P < 0.001, linear mixed model ANOVA with a Bonferroni post-hoc test). (C, F, and I) Percent changes from the 1st to the 25th injection for DA (black) and 5-HT (pink). The change in 5-HT peak current from the 1st to 25th injection was also significant for each device type using two-tailed, paired t-tests (CFME, P < 0.001), (C-BDDME, P < 0.05), and (FS-BDDME, P < 0.001). The CFME also showed a significant decrease in DA response, although it had the lowest percentage drop of 4.9 ± 1.5% (t-test, two-tailed, paired, P < 0.05).
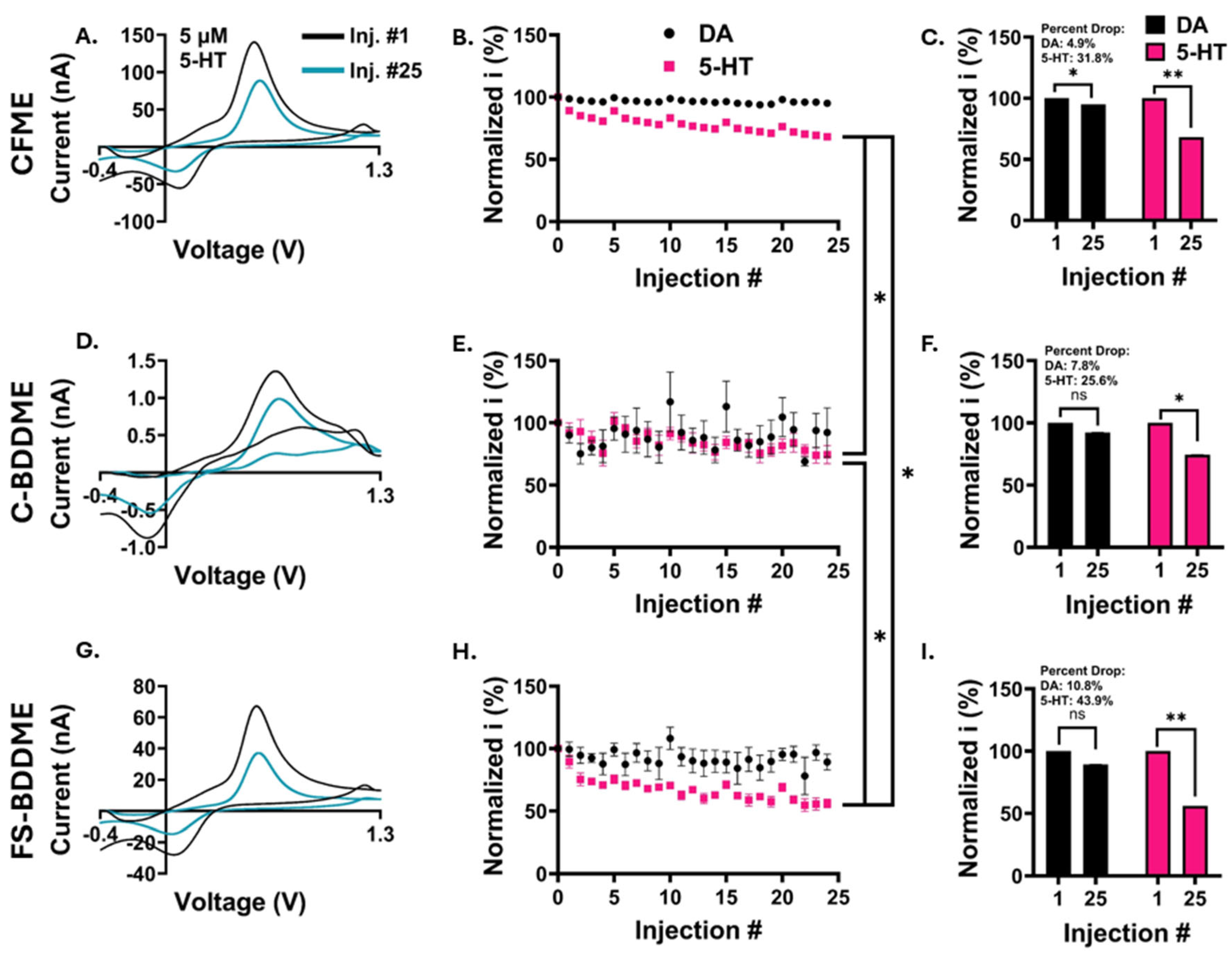
Figure 5.
Electrochemical fouling results for 50 µM DA and 5-HT. (A, D, and G) Representative (n=1) changes in CVs from the 1st (black) to the 25th (pink) 5-HT bolus injection. (B, E, and H) 25 consecutive oxidation peak currents from 50 µM DA (black) and 50 µM 5-HT (pink) (n=4, 4, and 2 from top to bottom). The 5-HT fouling trajectories were significantly different between all three electrode types (P < 0.001, linear mixed model ANOVA with a Bonferroni post-hoc test). (C, F, and I) Percent changes from the 1st to the 25th injection for DA (black) and 5-HT (pink). The change in serotonin peak current from the 1st to 25th injection was also significant for each device type using two-tailed, paired t-tests (CFME, P < 0.001), (C-BDDME, P < 0.001), and (FS-BDDME, P < 0.05).
Figure 5.
Electrochemical fouling results for 50 µM DA and 5-HT. (A, D, and G) Representative (n=1) changes in CVs from the 1st (black) to the 25th (pink) 5-HT bolus injection. (B, E, and H) 25 consecutive oxidation peak currents from 50 µM DA (black) and 50 µM 5-HT (pink) (n=4, 4, and 2 from top to bottom). The 5-HT fouling trajectories were significantly different between all three electrode types (P < 0.001, linear mixed model ANOVA with a Bonferroni post-hoc test). (C, F, and I) Percent changes from the 1st to the 25th injection for DA (black) and 5-HT (pink). The change in serotonin peak current from the 1st to 25th injection was also significant for each device type using two-tailed, paired t-tests (CFME, P < 0.001), (C-BDDME, P < 0.001), and (FS-BDDME, P < 0.05).
Figure 6.
Electrochemical stability analysis with response repeatability and etching stability. Oxidation peak currents are normalized to the 0-minute values. (A-C) show 5-HT response repeatability over a two-hour recording period with 5 µM 5-HT injections every 10 minutes. Both the CFME (A, +0.35 ± 0.98%, n = 3) and the C-BDDME (B, -3.69 ± 8.41%, n =3) exhibited stable repeatability with no significance difference between the 0-minute and 120-minute data points (paired, two-tailed t-test P > 0.05). However, the FS-BDDME (C, -20.63 ± 5.5%, n = 2) showed a near-significant reduction in current between the 0-minute and 120-minute 5-HT exposures (paired, two-tailed t-test P ≈ 0.06). (D-F) Changes in electrochemical backgrounds in Tris Buffer before and after exposure to the DA waveform at 60 Hz for 24 hours to simulate 6 days of constant recording. CFMEs (D) exhibit a growth in background, C-BDDMEs (E) have a very stable background with almost no change in size, and FS-BDDME (F) backgrounds are reduced.
Figure 6.
Electrochemical stability analysis with response repeatability and etching stability. Oxidation peak currents are normalized to the 0-minute values. (A-C) show 5-HT response repeatability over a two-hour recording period with 5 µM 5-HT injections every 10 minutes. Both the CFME (A, +0.35 ± 0.98%, n = 3) and the C-BDDME (B, -3.69 ± 8.41%, n =3) exhibited stable repeatability with no significance difference between the 0-minute and 120-minute data points (paired, two-tailed t-test P > 0.05). However, the FS-BDDME (C, -20.63 ± 5.5%, n = 2) showed a near-significant reduction in current between the 0-minute and 120-minute 5-HT exposures (paired, two-tailed t-test P ≈ 0.06). (D-F) Changes in electrochemical backgrounds in Tris Buffer before and after exposure to the DA waveform at 60 Hz for 24 hours to simulate 6 days of constant recording. CFMEs (D) exhibit a growth in background, C-BDDMEs (E) have a very stable background with almost no change in size, and FS-BDDME (F) backgrounds are reduced.
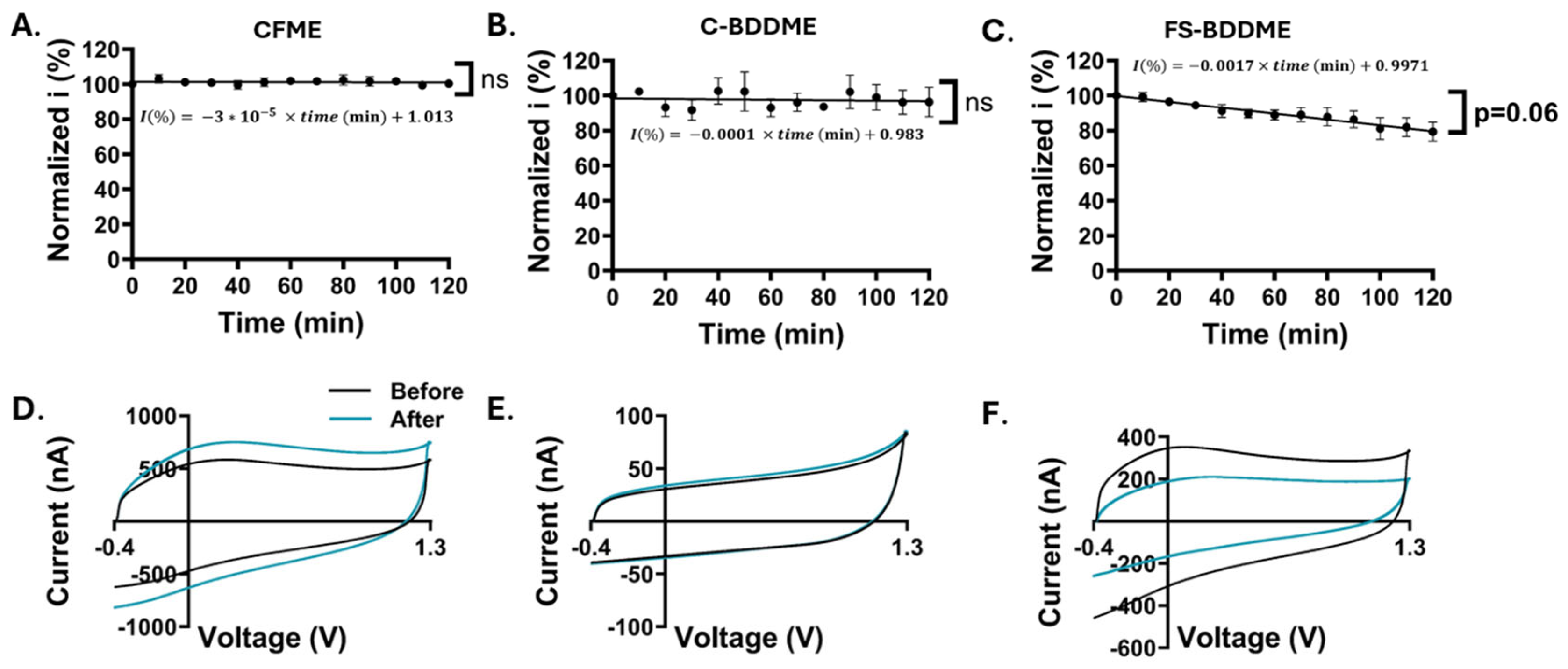