1. Introduction
Diabetic nephropathy (DN) is the leading cause of end-stage kidney disease and accumulating data show that damage of the glomerular filter resulting in proteinuria is one of the major mechanisms of renal impairment [
1,
2]. It is now widely acknowledged that within the glomerular filtration barrier, the podocytes play a pivotal role in controlling the passage of proteins into the urinary space [
3,
4]. and the onset of albuminuria in DN reflects diabetic podocytopathy [
5,
6].
Podocytes are unique, highly specialized terminally differentiated cells of epithelial origin found in the kidney glomeruli. Diverse biological as well as structural properties of podocytes make them the crucial component of renal filter [
7]. Apart from mechanical supporting the integrity of the glomerular tuft, podocytes are involved in the synthesis and repair of all components of the glomerular filter [
8,
9]. The cells are anchored to the glomerular basement membrane (GBM) by interdigitating foot processes. The gaps between the neighboring protrusions are bridged by slit diaphragms (SD) that constitute the final barrier for protein loss during filtration through the capillary wall. Podocyte structure, function, intercellular contact, as well as control of proteinuria are strictly associated with SD integrity [
10]. Despite their critical role in maintaining normal renal function, mature kidney podocytes have a limited ability to regenerate in response to injury, which results in permanent alterations in glomerular structure. This is why podocyte damage, detachment and loss are considered to be pivotal steps towards progressive chronic kidney disease [
11,
12].
The transforming growth factor-beta (TGF-β) family of pleiotropic cytokines consists of three (TGF-β1, TGF-β2 and TGF-β3) isoforms, of which TGF-β1 has been established as the predominant isoform expressed in the kidney [
13,
14]. In physiological conditions, TGF-β1 maintains tissue homeostasis by regulating a broad range of cellular processes and interactions of the cells with the extracellular environment [
15,
16]. However, excessive TGF-β activity is implicated in the pathogenesis of various diseases by contributing to changes in tissue structure, immunity, redox balance, motility of the cells and many other features [
17,
18]. Nearly all kidney diseases are associated with TGF-β1 upregulation, and in DN it plays a key role in the development of pathogenic changes in renal tissues [
19]. Moreover, many lines of evidence have shown that TGF-β1 is a central mediator of podocyte injury [
20,
21,
22]. In the diabetic kidney, various stimuli such as hyperglycemia, reactive oxygen species (ROS), angiotensin II (Ang II), thrombospondin-1 (TSP-1) and advanced glycation end products (AGEs) induce TGF-β1 synthesis and activate TGF-β1-dependent signalling, which results in diverse injurious changes underlying DN. Also, podocytes, the most vulnerable renal cells, become targets for the overactive TGF-β1 system. This results in a series of functional, morphological and phenotypic changes, leading to irreversible podocyte impairment and loss [
23]. To date, there is no cure for diabetic podocytopathy.
Urolithins, the dibenzo[
b,d]pyran-6-one derivatives, are polyphenol metabolites that are produced by the human gut microbiota from ellagitannins and ellagic acid-rich food products such as nuts, pomegranate and berries (
Figure 1). The family consists of several isoforms, of which urolithin A (UA) is the most abundant form in humans [
24]. Studies have shown that urolithins, and particularly UA, exhibit various biological activities including antioxidant, anticancer, anti-inflammatory and antiglycative properties [
25,
26]. The wide range of these beneficial effects is mediated by diverse urolithin-mediated intracellular mechanisms, such as modulation of apoptosis, signal transduction, cell cycle, gene expression, and others [
27]. However, detailed knowledge of the interactions between urolithins and systems regulating cell functions in different organs still remains incomplete. The presence of urolithins in urine indicates their direct contact with renal tissue. Nevertheless, only a few studies on the effects of urolithins in the kidney have been published so far. We have shown recently that UA improved the viability of podocytes exposed to high glucose and additionally, expression and cellular localization of nephrin, the central component of SD was modulated by this compound [
28]
In the present study, we aimed to investigate whether UA can ameliorate injury of podocytes under conditions mimicking diabetes by influencing the activity of TGF-β1.
4. Discussion
Presented in this study results demonstrate that urolithin A counteracts the phenotypic changes induced in podocytes by TGF-β1 and high glucose. We show that under conditions mimicking the diabetic milieu, UA suppresses podocyte motility, inhibits the Smad2-dependent TGF-β1 signalling and opposes the epithelial-to-mesenchymal transition.
Podocyte injury and loss lead to irreversible changes in the glomerular filtration barrier (GFB), making these cells crucial in the progression of diabetic kidney disease (DKD) [
57,
58]. Typically, podocyte impairment is manifested by effacement of foot processes which is associated with loss of SD components, dedifferentiation, EMT, and finally, detachment and loss of viable, apoptotic or necrotic cells [
59,
60,
61,
62].
EMT is a complex process mediating podocyte dysfunction in diabetes as well as in non-diabetic chronic kidney disease such as renal fibrosis or focal segmental glomerulosclerosis (FSGS) [
63,
64]. During EMT, epithelial features of podocytes, including nephrin expression, are lost and the cells acquire mesenchymal features that are manifested by increased migratory properties and expression of proteins such as fibronectin, α-smooth muscle actin and others [
65,
66]. The in vivo and in vitro studies show that among different microenvironmental stimuli, TGF-β is a potent inducer of EMT in podocytes under normal [
21,
50,
67], as well as under high glucose conditions [
68,
69]. High glucose concentration induces EMT in podocytes not only through activation of TGF-β signalling but also through several other molecular mechanisms [
49,
70,
71]. EMT is considered to be the major pathomechanism underlying podocytopenia in diabetic kidney [
65]
Our results indicate that TGF-β1 and HG, separately and in concert, induced in podocytes changes typical for EMT, which was abolished upon treatment with UA. Both these factors independently increased the ability of podocytes to migrate (
Figure 3A,B), which is consistent with previous reports [
48,
72]. Similarly, the expression of nephrin, the key component of SD and marker protein of podocytes, was separately downregulated by both these factors. However, the effect induced by TGF-β1 was significantly augmented in HG conditions (
Figure 2). On the other hand, expression of fibronectin was strongly increased by TGF-β1, as well as by high glucose (
Figure 4), and the effect was enhanced when podocytes were exposed to both factors simultaneously (
Figure 4A). In the presence of UA, the impact of TGF-β1 was apparently suppressed, while the changes elicited by prolonged exposure of the cells to HG were reversed.
In diabetic kidneys, hyperglycemic milieu and TGF-β act on podocytes simultaneously [
73]. Under HG conditions, increased interaction of podocytes with TGF-β results from overproduction and secretion by glomerular endothelial and mesangial cells of TGF-β and TGF-β mRNA-containing exosomes [
74]. Moreover, also podocytes can produce TGF-β acting in an autocrine manner, and this phenomenon occurs also in the normoglycemic milieu [
75,
76,
77]. In our in vitro experiments, most of the features investigated here were modified by TGF-β1 not only in HG but also in NG conditions, while high glucose concentration was an independent factor affecting the cells. However, UA hindered the activity of TGF-β1 and reversed the changes induced by prolonged preincubation of podocytes in HG.
In physiological conditions, podocytes display a limited ability to migrate, which allows them to withstand injurious stimuli such as inflammatory or mechanical stress [
7,
78,
79]. However, excessive stress leads to dysregulated cell motility which is tightly associated with disruption of SD, proteinuria and podocyte detachment [
79,
80]. We show here that in HG conditions and upon stimulation with TGF-β1, increased podocyte motility (
Figure 3B) was associated with the elevation of β3 integrin, whereas abolishment by UA of cell migration was paralleled by downregulation ofβ3 integrin (
Figure 3D,F). These results are consistent with previously published reports revealing that there is an inverse relationship between the expression of β3 integrin and the capability of podocytes to migrate [
48].
We demonstrate here that typical for EMT responses of podocytes to TGF-β1, such as the decrease of nephrin and the increase of integrin β3 or fibronectin were enhanced in the hyperglycemic milieu. In both NG and HG conditions, UA significantly opposed the detrimental effects of the cytokine, which was at least partly mediated by interrupting the TGF-β1 signal transduction. As shown in
Figure 5, high glucose concentration increased the expression of TβRI and TβRII receptors, which is consistent with previous reports [
81,
82,
83]. However, in the presence of UA, we have noted the rise in TβRI mRNA with a concomitant drop in protein expression. In our recent study, we noted a similar discrepancy between mRNA and protein during the quantification of UA-dependent modulation of nephrin expression [
28]. Such inconsistency is a frequent phenomenon [
84] and suggests that urolithin A could induce post-transcriptional changes in the expression of proteins. Upon treatment with TGF-β1, both TβRI and TβRII receptors were downregulated. Reports regarding the influence of TGF-β on its own receptors are conflicting and reveal up, as well as downregulation of TβRI and TβRII [
85,
86]. However, it has also been found that the final effect depends on the duration of exposure of the cells to TGF-β [
87]. Brief administration of TGF-β resulted in upregulation of its own receptors, whereas prolonged incubation reduced expression of TβRI and TβRII. In our experiments, the podocytes were exposed to TGF-β1 for 24 hours, corresponding the prolonged -incubation, which resulted in a decrease in the expression of receptors. Co-incubation of HG-treated podocytes with TGF-β1 along with UA further reduced the TβRI expression (
Figure 5B), which, most likely, contributed to diminished responsiveness of the TGF-β receptor system to stimulation by the ligand. Moreover, we show here that UA also interrupts the TGF-β1 signal transduction downstream to the receptors. TGF−β/Smad signalling pathway is one of the most important signal pathways mediating EMT and apoptosis in podocytes [
71,
88]. Formation of TGF-β/TβRII/TβRI complex triggers the downstream phosphorylation and activation of Smad2/3 proteins which is crucial for further transduction of the signal to the nucleus [Valcourt 2005]. We have proven, that UA reduces the mediated by HG and TGF-β1 increase of pSmad2/Smad2 ratio (
Figure 6B). In the presence of HG and TGF-β1, the expression of not only the phosphorylated form (pSmad2) but also of the unphosphorylated Smad2 was elevated (
Figure 6C,D). Yet, in HG conditions, UA stimulated an even bigger increase of Smad2, which was accompanied by strong suppression of pSmad2 expression.
Detailed mechanisms by which UA opposes the effects of high glucose and TGF-β remain to be established. It is known so far that UA is capable of regulating the expression of various proteins by modulating transcription and post-transcriptional processes [
29,
89,
90]. The broad range of beneficial biological activities exerted by urolithins, first of all by UA, has been listed lately in the comprehensive review by Hasheminezhad et al. [
27]. Recently, inhibition by UA of EMT in cancer cells [
90,
91] and inhibition of TGF-β signalling in renal epithelial cells [
92] were reported. However, relatively few research results refer to the influence of UA on renal disorders, with only a few publications discussing its impact on podocytes. Our results reveal that in conditions mimicking diabetes, UA inhibits the EMT-associated changes in podocytes. Moreover, we show here that also in the normoglycemic milieu, UA opposes the effects of TGF-β1, the principal mediator in the development of kidney fibrosis, glomerulosclerosis and CKD [
13,
93,
94]
Figure 1.
Ellagitannins (ET) and ellagic acid (EA) are naturally occurring polyphenolic bioactive compounds found in fruits and seeds of various food plants. ET are hydrolyzed to EA in the upper part of the gastrointestinal tract and further converted by microbiota in the large intestine into urolithins. Depending on individual microbiota composition, various urolithin isoforms are produced, of which urolithin A is the most common form [
29]. In contrast to ET and EA, urolithins are easily absorbed in the gut.
Figure 1.
Ellagitannins (ET) and ellagic acid (EA) are naturally occurring polyphenolic bioactive compounds found in fruits and seeds of various food plants. ET are hydrolyzed to EA in the upper part of the gastrointestinal tract and further converted by microbiota in the large intestine into urolithins. Depending on individual microbiota composition, various urolithin isoforms are produced, of which urolithin A is the most common form [
29]. In contrast to ET and EA, urolithins are easily absorbed in the gut.
Figure 2.
The effect of UA on the TGF-β1 and HG-induced downregulation of nephrin. (A, C) Quantitative flow cytometry analysis of UA effect on nephrin expression at the podocyte surface (A) and total nephrin (C). Podocytes cultured for 7d in normal (5.5 mM, NG) or high (25 mM, HG) glucose were incubated for 24 h with 5 ng/ml TGF-β1 and 10 µM UA, stained with phycoerythrin-conjugated antibody against the extracellular nephrin domain (A) or total nephrin (C) and analyzed by flow cytometry. (B, D) Representative histograms showing the effect of UA on surface (B) and total (D) nephrin expression. (E) Quantitative confocal microscopy analysis of total nephrin expression. (F) Representative confocal microscopy images of immunofluorescent staining against nephrin. Results show mean ± SEM. Student’s t-test and ANOVA test were used to calculate p values. For (A) and (C) *p < 0.05 vs. NG Control, ** p < 0.05 vs. respective TGF-β1 and Control, *** p<0.001 vs. NG Control, # p<0.01 vs. respective TGF-β1, & p<0.05 vs. HG Control, @ p<0.05 vs.NG TGF-β1 (n =3- 5). For (E) *p<0.001 vs, NG Control, ** p<0.001 vs. HG Control, & p<0.001 vs. respective TGF-β1, # p<0.05 vs. HG Control. 553 cells were analyzed in two independent experiments. MFI: mean fluorescence intensity.
Figure 2.
The effect of UA on the TGF-β1 and HG-induced downregulation of nephrin. (A, C) Quantitative flow cytometry analysis of UA effect on nephrin expression at the podocyte surface (A) and total nephrin (C). Podocytes cultured for 7d in normal (5.5 mM, NG) or high (25 mM, HG) glucose were incubated for 24 h with 5 ng/ml TGF-β1 and 10 µM UA, stained with phycoerythrin-conjugated antibody against the extracellular nephrin domain (A) or total nephrin (C) and analyzed by flow cytometry. (B, D) Representative histograms showing the effect of UA on surface (B) and total (D) nephrin expression. (E) Quantitative confocal microscopy analysis of total nephrin expression. (F) Representative confocal microscopy images of immunofluorescent staining against nephrin. Results show mean ± SEM. Student’s t-test and ANOVA test were used to calculate p values. For (A) and (C) *p < 0.05 vs. NG Control, ** p < 0.05 vs. respective TGF-β1 and Control, *** p<0.001 vs. NG Control, # p<0.01 vs. respective TGF-β1, & p<0.05 vs. HG Control, @ p<0.05 vs.NG TGF-β1 (n =3- 5). For (E) *p<0.001 vs, NG Control, ** p<0.001 vs. HG Control, & p<0.001 vs. respective TGF-β1, # p<0.05 vs. HG Control. 553 cells were analyzed in two independent experiments. MFI: mean fluorescence intensity.
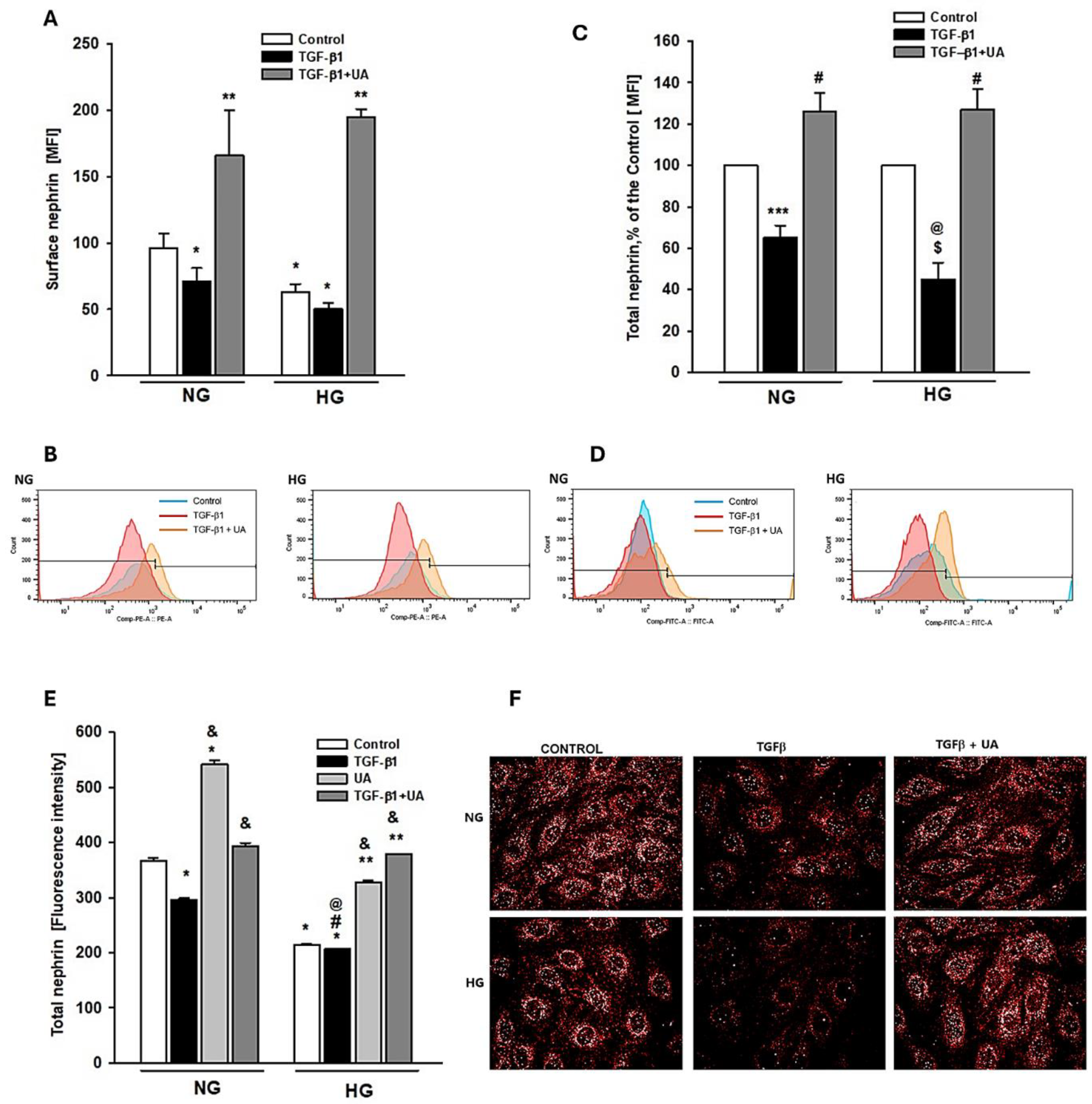
Figure 3.
Effects of UA on migratory capacity and expression of β3 integrin in podocytes exposed to TGF-β1 and HG. Podocytes cultured for 7d in normal (5.5 mM, NG) or high (25 mM, HG) glucose were incubated for 24 h with 5 ng/ml TGF-β1 and/or 10 µM UA. (A) Representative image of the wound healing test. After making a scratch in the cell monolayer (time 0), the podocytes were incubated in indicated media for 24 hours (B) Quantification of wound healing assay (n=4). (C) Representative immunoblot for integrin β3 expression. 30-µg protein samples from total cell lysates were subjected to Western blot analysis followed by quantitative densitometric analysis. (D) Quantification of Western blot analyses of β3 integrin expression (n=3). (E) Representative confocal microscopy images of immunofluorescent staining against β3 integrin. (F) Quantitative confocal microscopy analysis of β3 integrin expression. 754 cells were analyzed in two independent experiments. Results show mean ± SEM. Student’s t-test, Mann-Whitney U test and ANOVA were used to calculate p values. *p<0.001 vs. NG Control, ** p<0.001 vs. HG Control, # p<0.001 vs. respective TGF-β1, @ p<0.001 vs, NG TGF-β1.
Figure 3.
Effects of UA on migratory capacity and expression of β3 integrin in podocytes exposed to TGF-β1 and HG. Podocytes cultured for 7d in normal (5.5 mM, NG) or high (25 mM, HG) glucose were incubated for 24 h with 5 ng/ml TGF-β1 and/or 10 µM UA. (A) Representative image of the wound healing test. After making a scratch in the cell monolayer (time 0), the podocytes were incubated in indicated media for 24 hours (B) Quantification of wound healing assay (n=4). (C) Representative immunoblot for integrin β3 expression. 30-µg protein samples from total cell lysates were subjected to Western blot analysis followed by quantitative densitometric analysis. (D) Quantification of Western blot analyses of β3 integrin expression (n=3). (E) Representative confocal microscopy images of immunofluorescent staining against β3 integrin. (F) Quantitative confocal microscopy analysis of β3 integrin expression. 754 cells were analyzed in two independent experiments. Results show mean ± SEM. Student’s t-test, Mann-Whitney U test and ANOVA were used to calculate p values. *p<0.001 vs. NG Control, ** p<0.001 vs. HG Control, # p<0.001 vs. respective TGF-β1, @ p<0.001 vs, NG TGF-β1.
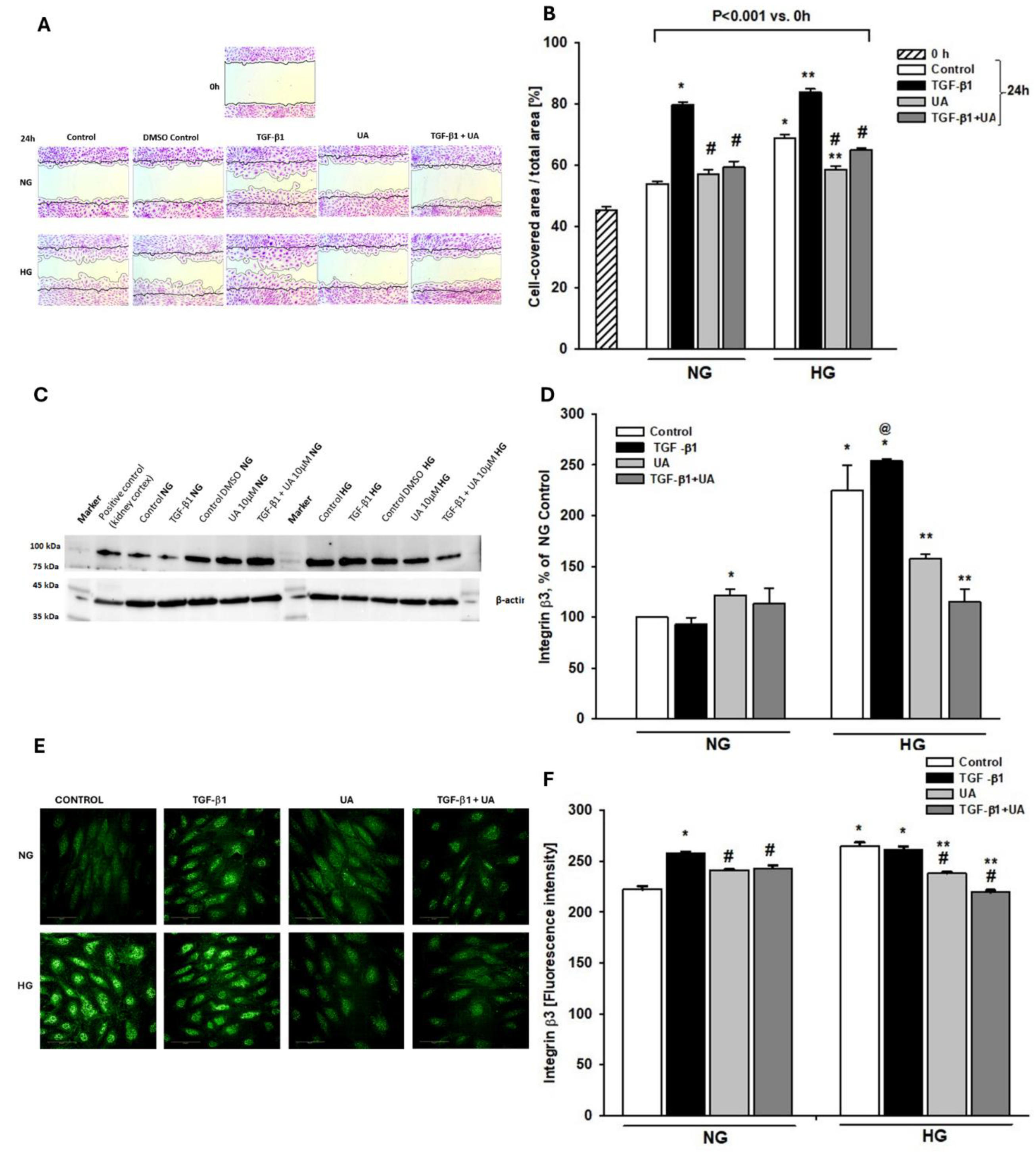
Figure 4.
Modulation by UA of fibronectin expression in podocytes exposed to TGF-β1 and HG. Podocytes cultured for 7d in normal (5.5 mM, NG) or high (25 mM, HG) glucose were incubated for 24 h with 5 ng/ml TGF-β1 and/or 10 µM UA. (A) Results of quantitative RT-PCR analysis for fibronectin. Relative levels of mRNA were normalized to β-actin (n=4). (B) Quantification of Western blot analyses of fibronectin expression (n=3-4). (C) Representative immunoblot for fibronectin expression. 30-µg protein samples from total cell lysates were subjected to Western blot analysis followed by quantitative densitometric analysis. (D) Representative confocal microscopy images of immunofluorescent staining showing fibronectin (red), F-actin (green) and counterstained woth DAPI (blue) . (E) Quantitative confocal microscopy analysis of fibronectin expression. 758 cells were analyzed in two independent experiments. Results show mean ± SEM. Student’s t-test, Mann-Whitney U test and ANOVA test were used to calculate p values. For (A) *p<0.001 vs. NG Control, **p<0.01 vs. respective Control, @p<0.01 vs. NG TGF-β1. For (B) *p<0.01 vs.NG Control,**p<0.02 vs. respective TGF-β1, #p<0.02 vs. HG Control. For (E) *p<0.001 vs. respective Control and TGF-β1, **p<0.001 vs. HG Control.
Figure 4.
Modulation by UA of fibronectin expression in podocytes exposed to TGF-β1 and HG. Podocytes cultured for 7d in normal (5.5 mM, NG) or high (25 mM, HG) glucose were incubated for 24 h with 5 ng/ml TGF-β1 and/or 10 µM UA. (A) Results of quantitative RT-PCR analysis for fibronectin. Relative levels of mRNA were normalized to β-actin (n=4). (B) Quantification of Western blot analyses of fibronectin expression (n=3-4). (C) Representative immunoblot for fibronectin expression. 30-µg protein samples from total cell lysates were subjected to Western blot analysis followed by quantitative densitometric analysis. (D) Representative confocal microscopy images of immunofluorescent staining showing fibronectin (red), F-actin (green) and counterstained woth DAPI (blue) . (E) Quantitative confocal microscopy analysis of fibronectin expression. 758 cells were analyzed in two independent experiments. Results show mean ± SEM. Student’s t-test, Mann-Whitney U test and ANOVA test were used to calculate p values. For (A) *p<0.001 vs. NG Control, **p<0.01 vs. respective Control, @p<0.01 vs. NG TGF-β1. For (B) *p<0.01 vs.NG Control,**p<0.02 vs. respective TGF-β1, #p<0.02 vs. HG Control. For (E) *p<0.001 vs. respective Control and TGF-β1, **p<0.001 vs. HG Control.
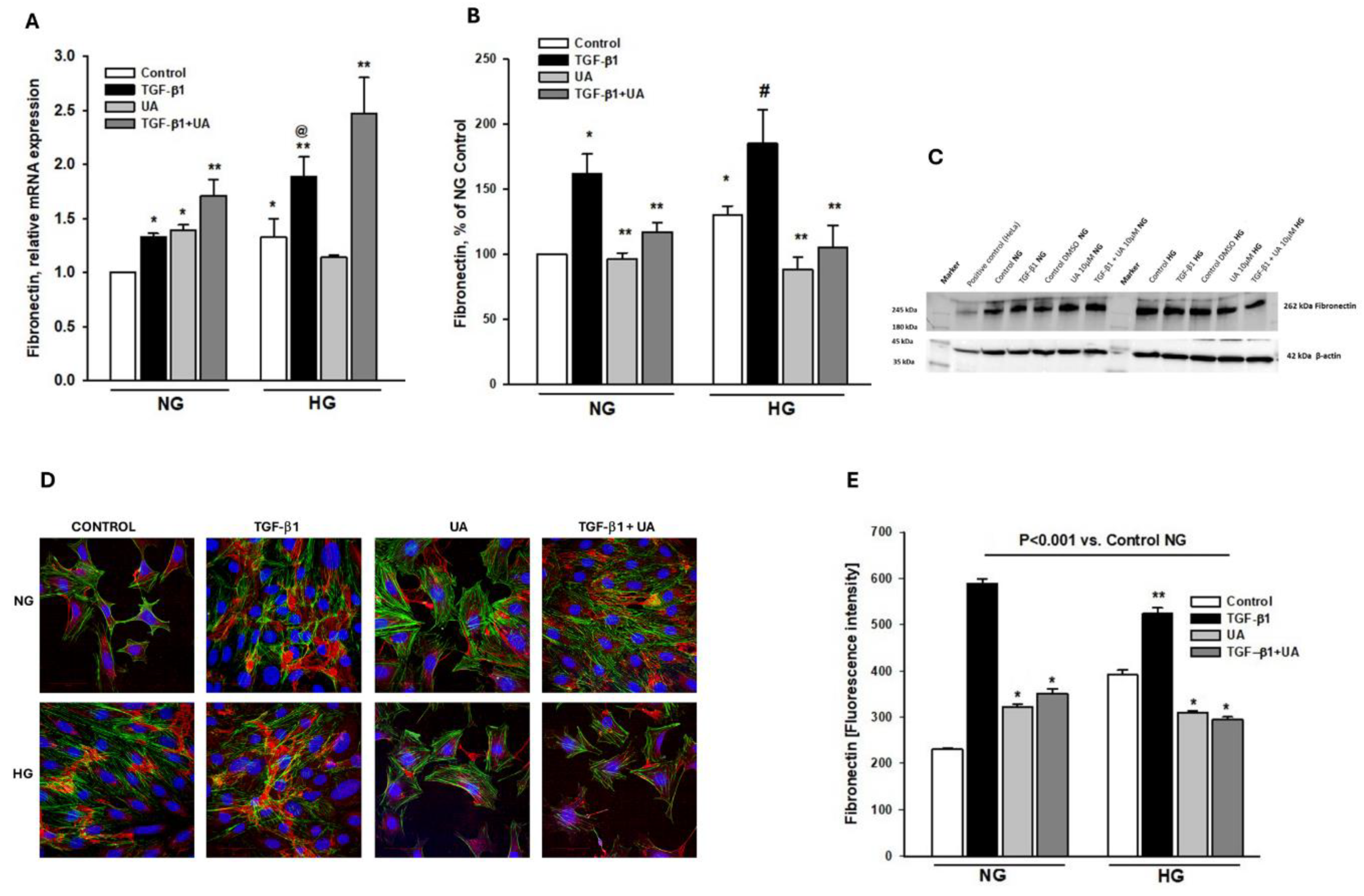
Figure 5.
Effects of UA on the expression of TGF-β1 receptors TβRI and TβRII. Podocytes cultured for 7d in normal (5.5 mM, NG) or high (25 mM, HG) glucose were incubated for 24 h with 5 ng/ml TGF-β1 and/or 10 µM UA. (A) Results of quantitative RT-PCR analysis for TβRI. Relative levels of mRNA were normalized to β-actin (n=3). (B) Quantitative confocal microscopy analysis of TβRI expression. 810 cells were analyzed in two independent experiments. (C) Representative confocal microscopy images of immunofluorescent staining against TβRI. (D) Results of quantitative RT-PCR analysis for f TβRII. Relative levels of mRNA were normalized to β-actin (n=3). (E) Quantitative confocal microscopy analysis of TβRII expression. 840 cells were analyzed in two independent experiments. (F) Representative confocal microscopy images of immunofluorescent staining against TβRII. Results show mean ± SEM. Student’s t test and ANOVA test were used to calculate p values. For (A) *p<0.05 vs. respective Control, **p<0.001 vs. respective Control, ***p<0.005 vs. NG Control. For (B) *p<0.001 vs. respective Control, **p<0.001 vs. NG Control, #p<0.001 vs. TGF-β1, @p<0.001 vs. NG TGF-β1. For (D) *p<0.05 vs. respective Control, #p<0.01vs. UA. For (E) *p<0.001 vs. NG Control, ** p<0.001 vs. HG Control, #p<0.001 vs. respective TGF-β1 and UA, @p<0.001 vs NG TGF-β1.
Figure 5.
Effects of UA on the expression of TGF-β1 receptors TβRI and TβRII. Podocytes cultured for 7d in normal (5.5 mM, NG) or high (25 mM, HG) glucose were incubated for 24 h with 5 ng/ml TGF-β1 and/or 10 µM UA. (A) Results of quantitative RT-PCR analysis for TβRI. Relative levels of mRNA were normalized to β-actin (n=3). (B) Quantitative confocal microscopy analysis of TβRI expression. 810 cells were analyzed in two independent experiments. (C) Representative confocal microscopy images of immunofluorescent staining against TβRI. (D) Results of quantitative RT-PCR analysis for f TβRII. Relative levels of mRNA were normalized to β-actin (n=3). (E) Quantitative confocal microscopy analysis of TβRII expression. 840 cells were analyzed in two independent experiments. (F) Representative confocal microscopy images of immunofluorescent staining against TβRII. Results show mean ± SEM. Student’s t test and ANOVA test were used to calculate p values. For (A) *p<0.05 vs. respective Control, **p<0.001 vs. respective Control, ***p<0.005 vs. NG Control. For (B) *p<0.001 vs. respective Control, **p<0.001 vs. NG Control, #p<0.001 vs. TGF-β1, @p<0.001 vs. NG TGF-β1. For (D) *p<0.05 vs. respective Control, #p<0.01vs. UA. For (E) *p<0.001 vs. NG Control, ** p<0.001 vs. HG Control, #p<0.001 vs. respective TGF-β1 and UA, @p<0.001 vs NG TGF-β1.
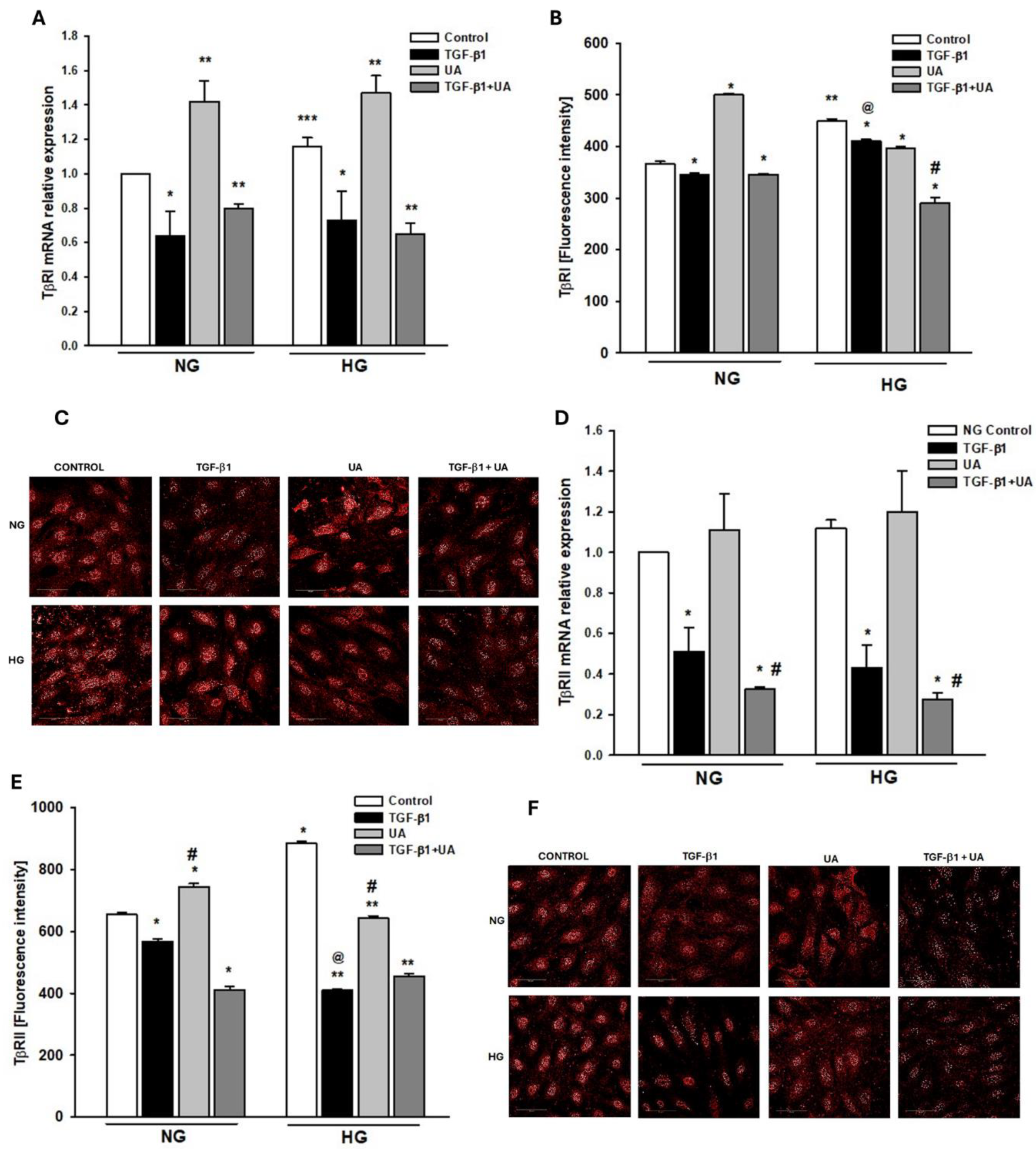
Figure 6.
Effects of UA on Smad2-dependent signalling of TGF-β1. Podocytes cultured for 7d in normal (5.5 mM, NG) or high (25 mM, HG) glucose were incubated for 24 h with 5 ng/ml TGF-β1 and/or 10 µM UA. (A) Representative immunoblots for expression of Smad2 and phospho-Smad2 (pSmad2). 30-µg protein samples from total cell lysates were subjected to Western blot analysis followed by quantitative densitometric analysis. (B) Quantification of Western blot analyses of pSmad2/Smad2 expression ratio (n=3). (C) Quantitative confocal microscopy analysis of Smad2 expression. 550 cells were analyzed in two independent experiments. (D) Quantitative confocal microscopy analysis of pSmad2 expression. 550 cells were analyzed in two independent experiments. (E) Representative confocal microscopy images of immunofluorescent staining against Smad2. (F) Representative confocal microscopy images of immunofluorescent staining against pSmad2. Results show mean ± SEM. Student’s t-test and ANOVA test were used to calculate p values. For (B) *p<0.001 vs. NG Control, **p<0.03 vs. HG Control, ***p<0.01 vs. NG TGF-b1, #p<0.03 vs. HG TGF-b1. For (C) *p<0.001 vs. NG Control, ** p<0.01 vs. HG Control, ***p<0.001 vs. Control and TGF-b1. For (D) *p<0.001 vs. NG Control, **p<0.001 vs. respective Control, #p<0.01 vs. respective TGF-b1.
Figure 6.
Effects of UA on Smad2-dependent signalling of TGF-β1. Podocytes cultured for 7d in normal (5.5 mM, NG) or high (25 mM, HG) glucose were incubated for 24 h with 5 ng/ml TGF-β1 and/or 10 µM UA. (A) Representative immunoblots for expression of Smad2 and phospho-Smad2 (pSmad2). 30-µg protein samples from total cell lysates were subjected to Western blot analysis followed by quantitative densitometric analysis. (B) Quantification of Western blot analyses of pSmad2/Smad2 expression ratio (n=3). (C) Quantitative confocal microscopy analysis of Smad2 expression. 550 cells were analyzed in two independent experiments. (D) Quantitative confocal microscopy analysis of pSmad2 expression. 550 cells were analyzed in two independent experiments. (E) Representative confocal microscopy images of immunofluorescent staining against Smad2. (F) Representative confocal microscopy images of immunofluorescent staining against pSmad2. Results show mean ± SEM. Student’s t-test and ANOVA test were used to calculate p values. For (B) *p<0.001 vs. NG Control, **p<0.03 vs. HG Control, ***p<0.01 vs. NG TGF-b1, #p<0.03 vs. HG TGF-b1. For (C) *p<0.001 vs. NG Control, ** p<0.01 vs. HG Control, ***p<0.001 vs. Control and TGF-b1. For (D) *p<0.001 vs. NG Control, **p<0.001 vs. respective Control, #p<0.01 vs. respective TGF-b1.
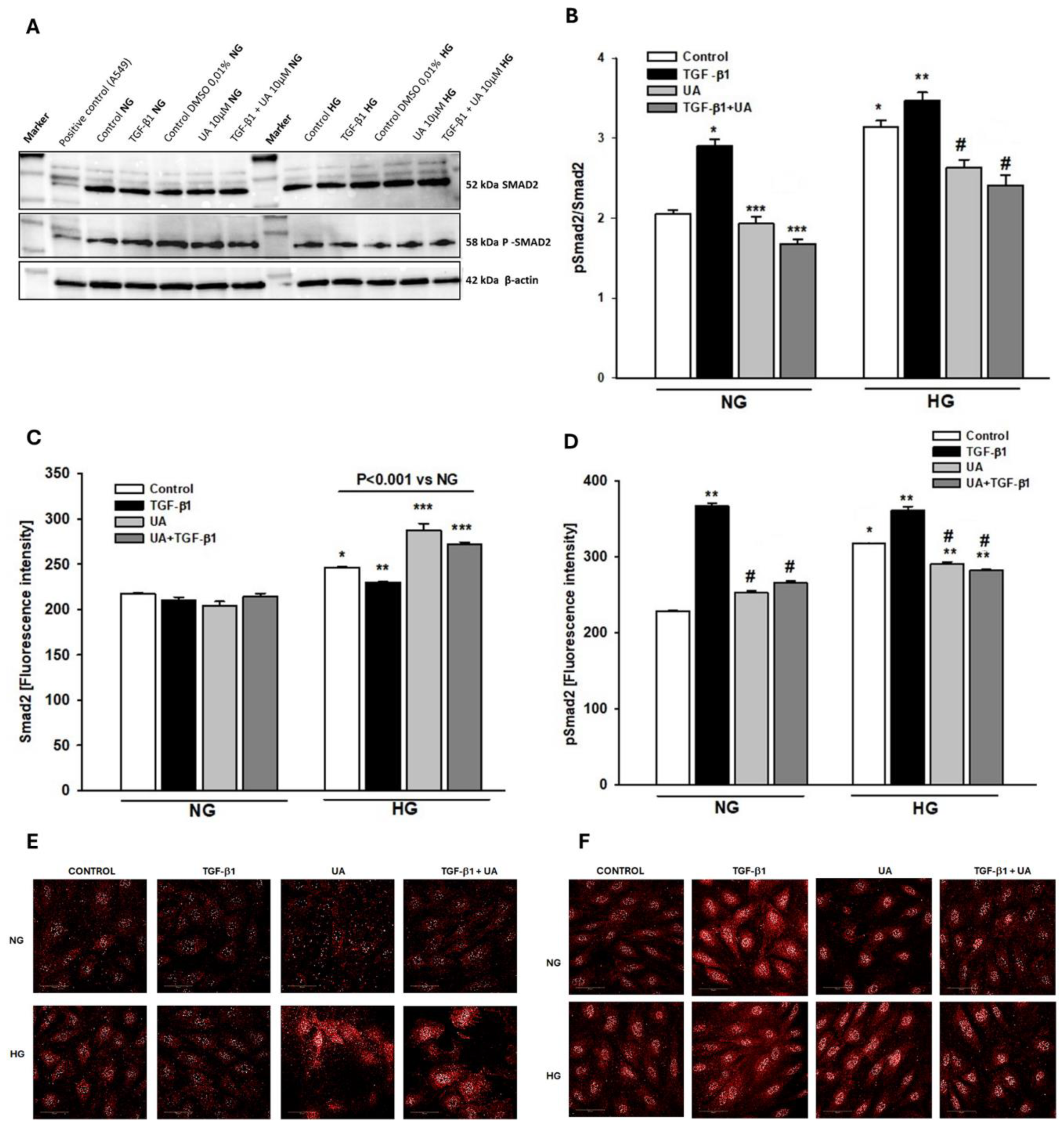